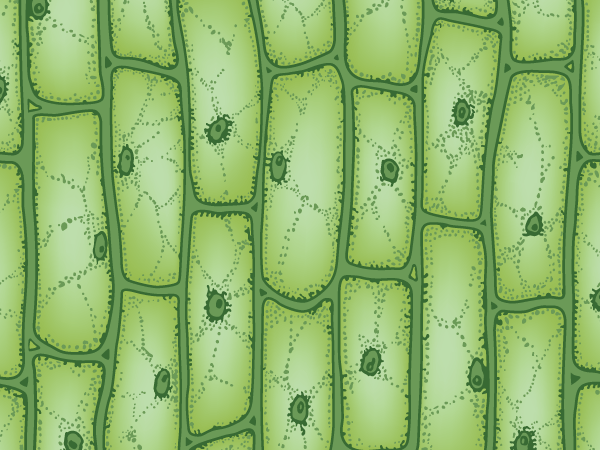
Deep dive: Cultivated meat scaffolding
Learn about scaffolding materials and methods for cultivated meat.
Scaffolding basics
Introduction
The range of meat products on the market exists on a spectrum of structural sophistication. On one end of the spectrum, there are less sophisticated processed products such as surimi and hot dogs; in the middle, minced products such as burgers and sausages; and on the other end, filets and steaks. In order to replicate the more sophisticated products, scientists will need to borrow and improve upon technologies from tissue engineering, regenerative medicine, and biomaterials science to recreate the complex multicellular architecture of meat. A review of the core bioengineering biology, scaffolding technologies, and methods to consider for the creation of complex structures are discussed below.
The extracellular matrix and mechanotransduction
The initial proliferation of stem cells is an essential part of the cultivated meat production process. How are trillions of stem cells then turned into structured meat via differentiation? In order to understand, background on the extracellular matrix and mechanotransduction is necessary (Ahmad et al. 2021).
In vivo, cells exist within a complex matrix of secreted proteins and proteoglycans called the extracellular matrix (ECM). The ECM has an inherent stiffness that in turn can influence cellular activity via specialized cell membrane proteins called integrins. Integrins act as mechanosensors to mediate downstream effector proteins, leading to the formation of focal adhesion complexes that connect the actomyosin cytoskeleton to the extracellular matrix. The integrin-mediated focal adhesion can be thought of as the midpoint of a tug-of-war between the ECM and a cell’s internal cytoskeleton. These connections collectively mediate a cell’s ability to sense the extracellular environment, leading to downstream signaling that can affect cell polarity, migration, and differentiation (Handorf et al., 2015; Sun et al., 2016); Dyachuk 2013; Odintsova et al. 2010). Altogether, this process is referred to as mechanotransduction (Figure 1).
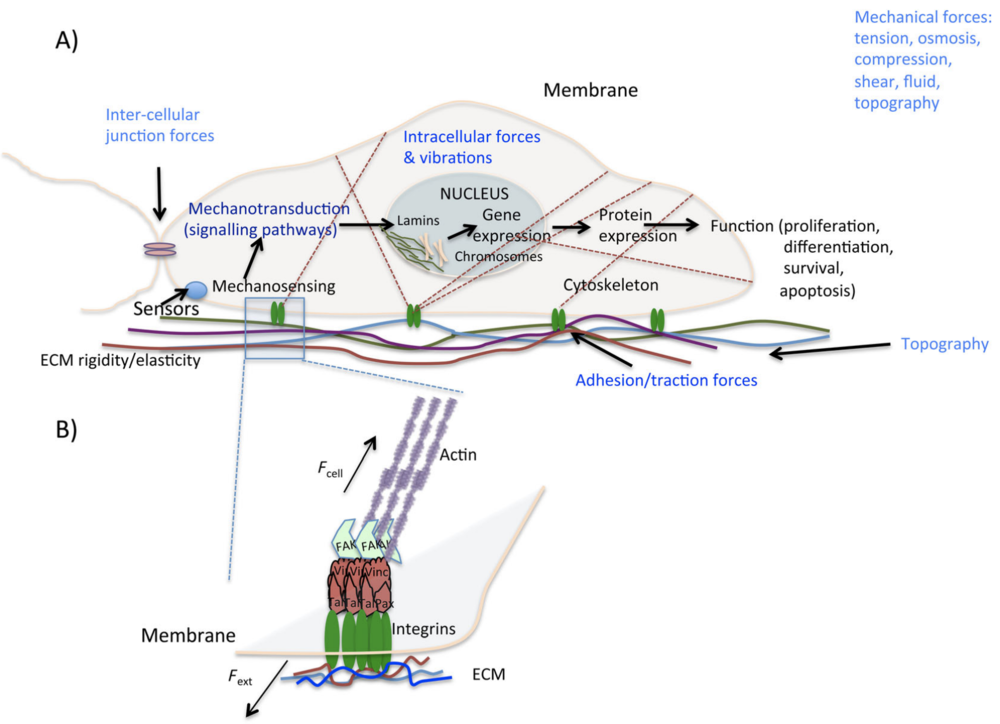
During embryogenesis and development, cells multiply and become specialized (i.e. differentiate) in part via cues from their extracellular environment. During this time, ECM components and cells themselves are actively in motion as they pass through key developmental landmarks such as the formation of the primitive streak, gastrulation, and tissue specialization (Loganathan et al., 2016). Additional factors such as ECM density and gradients, composition, and 3D topography can have large effects on cell behaviors (Rozario and DeSimone, 2010). In essence, as cells become defined, their gene expression patterns dictate the creation and secretion of specified ECM components, which in turn act as a feedback mechanism to further dictate cellular differentiation and migration, a process referred to as “dynamic reciprocity” (Bissell et al., 1982). Because components of the early embryo are all stem cells, this means that stem cells are exquisitely sensitive to ECM cues. Indeed, the regulation and maintenance of stem cells in the adult body is highly dependent on the ECM components that make up the stem cell niche, where each tissue’s niche typically contains a unique set of ECM components (Gattazzo et al., 2014).
Over the last two decades, a large set of studies have demonstrated the critical role of the ECM in the regulation of stemness and differentiation across all of the relevant cell types for cultivated meat production: pluripotent stem cells (Wang et al., 2015), mesenchymal stem cells (Engler et al., 2006), satellite cells (Calve et al., 2010), and adipogenic stem cells (Guneta et al., 2016). The importance of ECM proteins for myogenic differentiation has also been demonstrated in primary cultures from mussel (Dyachuk 2013; Odintsova et al. 2010). By mimicking in vitro the ECM stiffness and protein composition of a specified stem cell niche microenvironment, stem cells can be guided into predictable lineages. This biology, together with changes in the cell culture medium composition, will assist in driving the future creation of structured, 3D cultivated meat products.
Microcarriers
During the proliferation phase, anchorage-dependent stem cells are to be grown in bioreactors at large scales. As discussed in Bioprocessing, this non-native environment can lead to anoikis if cells are not adapted to suspension growth, grown as spheroids, or otherwise tricked into anchorage independence via small molecules such as Rho Kinase (ROCK) inhibitors. One commonly used method to bypass activation of anoikis in suspension-based bioreactors is through the use of microcarriers. Microcarriers are small bead-like structures typically 100-400µm in diameter (can be made larger) that permit attachment of cells via mimicry of ECM characteristics such as stiffness, topography, and porosity (discussed later, McKee and Chaudhry, 2017; Rafiq et al., 2013). Microcarriers are advantageous in bioreactor culture because they provide a large surface area to volume ratio, permitting high densities of cells relative to 2D culture and are able to be used in flexible (e.g. batch, fed-batch, perfusion) and controllable bioprocessing pipelines. Expansion of cells using microcarriers is relatively straightforward, either by the addition of more microcarriers where cells undergo bead-to-bead transfer (Verbruggen et al., 2018), or via enzymatic dissociation and passaging of cells to larger vessels via a seed train process (Oh et al., 2009; Rafiq et al., 2013). Furthermore, differentiation can occur on the microcarriers themselves (Park et al., 2014; Torgan et al., 2000),triggered by changes in media components, microcarrier characteristics, or shear forces via mechanotransduction (Jossen et al., 2014). Thus, microcarriers offer immense potential in scaling relevant stem cell populations and for providing differentiated cell types for cultivated meat production by leveraging the bioengineering principles discussed throughout.
Microcarriers can be modified in many ways for bioprocess optimization. For instance, microcarrier topography can be designed with nooks to prevent shear stress and patterned topography to improve cell alignment and polarity (Wu et al. 2018b). A recently-described method used a combination of oil-in-water emulsions followed by embossing during the crosslinking process to produce edible, grooved microcarriers for cultivated meat scale-up (Norris et al. 2022). Under the conditions tested, the performance of grooved and smooth microcarriers in supporting proliferation and myogenesis was approximately equivalent, but in theory such approaches could be useful for improving muscle cell alignment, differentiation, and maturation. The specific gravity of a microcarrier can influence its performance in a bioreactor, as denser and heavier microcarriers require more power to maintain in suspension culture, which in turn can influence the amount of shear force cells are subjected to. They are typically made from materials such as polystyrene, polyacrylamide, glass, or dextran, but can also be made from other plant-derived biomaterials (discussed later) or materials that can be enzymatically dissolved or degraded chemically, mechanically, or biologically (Rodrigues et al. 2019). Degradation methods, if selected, must be rapid and compatible with cell growth or the bioprocess parameters and while several materials and candidate methods exist, more optimization is needed (Bodiou et al. 2020). The microcarriers are themselves often coated with ECM proteins, given a positive charge, or chemically modified to be more hydrophilic to assist in cell attachment (Bodiou et al. 2020). Other strategies exist that play similar cell expansion roles as microcarriers, such as thermo-responsive nanobridges that are conjugated to ECM proteins (Harkness et al. 2019). These permit growth of cells in aggregates of a controlled size, which can later be dissociated and passaged in a cyclical nature following temperature-induced degradation and re-assembly. This strategy avoids problems associated with necrotic cores of large cellular aggregates due to lack of oxygen and nutrient availability as well as providing rapid and reversible degradation potential. A thermoreversible or sacrificial biomaterial (discussed later) may therefore yield more practical scale-up solutions.
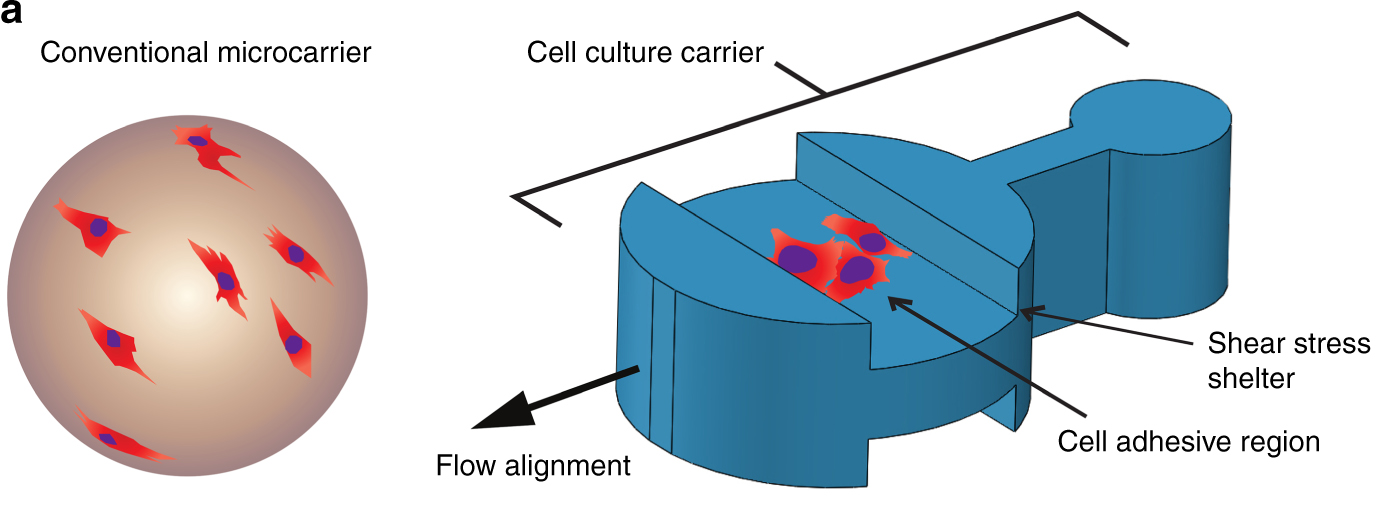
A variety of methods are available for downstream separation of cells from microcarriers following the proliferation phase (Mawji et al. 2022). However, the need for a separation step introduces additional costs—and potential food safety issues—that may be unacceptable for cultivated meat. A potential solution is the use of edible microcarriers that are incorporated into the final product (Bodiou et al. 2020). GFI grantee Dr. Marcel Machluf’s group demonstrated this concept using microcarriers made from a combination of collagen and chitosan, which were capable of supporting the proliferation of mouse, rabbit, sheep, and bovine cells (Zernov et al. 2022).
Heat-inactivated fungal pellets from Aspergillus oryzae were also shown to support the adherence and proliferation of C2C12 cells, with comparable growth to Cytodex 3, providing an animal component-free option (Ogawa et al. 2022). Spherical or grooved gelatin microcarriers (Norris et al. 2022) and commercially-available porous gelatin microcarriers (Liu et al. 2022b) were recently shown to support proliferation and differentiation of C2C12 cells and, in the latter case, 3T3-L1 preadipocytes and primary porcine satellite cells. Both of these studies demonstrated the formation of small-scale cultivated meat prototypes simply by aggregating the cell-laden microcarriers. Alginate microcarriers coated with gelatin supported proliferation of C2C12, 3T3-L1, primary porcine satellite, and commercially-available chicken satellite cells as well as differentiation of C2C12 cells (Kong et al. 2022). A patent related to edible microcarriers has been assigned to Modern Meadow (Marga et al. 2017). Such strategies could bypass the need to separate the cells from the microcarriers, which could be optimized for nutrition and flavor.
Further optimization of microcarriers for use in cultivated meat is still needed. For example, it is unlikely that a universal microcarrier can be developed for the range of cell types (discussed in Cell Lines) and species to be used. Most commercially available microcarriers were not designed for cultivated meat, though as of the last few years, this is beginning to change. Companies like Matrix F.T., Cellivate, CytoNiche, and Smart MCs produce edible microcarriers, and Tantti produces dissolvable microcarriers, with cultivated meat among the intended applications. Still, additional novel engineering will likely be necessary, similar to creating novel bioreactor designs. The seeding density, which can influence attachment and growth, will also need to be optimized for each cell type and microcarrier pair (Bodiou et al. 2020). A recent study explored the effects of seeding density and other process parameters on microcarrier-based culture of chicken myoblasts and identified distinct sets of conditions that promoted proliferation and differentiation (Yang et al. 2022). Such optimization efforts may be aided by the use of computational models to help identify the parameters—and interactions between parameters—whose optimization is most likely to positively affect the overall productivity of a given bioprocess (Camphuijsen et al. 2022).
Decisions on the use of microcarriers will need to be balanced against the intended product, which may influence whether microcarriers are used temporarily for proliferation, are degraded or dissolved during processing, or are edible and incorporated into a final product (Bodiou et al., 2020).
Scaffolding biomaterials
To produce structured and thick meat products, it is often helpful to grow cells on a scaffold. A scaffold ideally permits the attachment, differentiation, and maturation of cells in a specified manner, mimicking the 3D cytoarchitecture of meat while allowing for continuous perfusion of media, analogous to the vascularization of real tissue (discussed later). Therefore, considerations for the porosity of the scaffold, mechanical properties, and biocompatibility are paramount. In some cases, the production of differentiated cells on edible microcarriers (Marga et al., 2017, or other methods discussed later) may be chosen for inclusion as blended additives in a variety of unstructured products. However, fully replacing conventional animal meats will require replication of structured products such as steaks, requiring the skills of tissue engineers, materials science engineers, and other interdisciplinary scientists for building the methods for these applications.
How can we categorize scaffolds?
Scaffolds come in many different forms, and categorizing them can get a bit complicated. The infographic linked below describes one possible scheme for categorizing scaffolds based on their structure or function and the material(s) they’re made of. Gray outlined items indicate scaffolds belonging to more than one category. Scaffolds that have been tested with cell types or cells from species relevant to cultivated meat are indicated with icons. The “plant or fungus-based” category also includes molecules commonly produced by these groups that are also sourced from other organisms (e.g., cellulose from bacteria or algae,or chitosan from crustaceans). Adapted from Bomkamp et al. (2022) under the terms of the CC BY license.
The biomaterial that makes up a scaffold dictates many of its downstream properties. Biomaterials can be categorized broadly as natural, synthetic, or composite materials consisting of both natural and synthetic materials. Natural biomaterials include those that make up the native vertebrate ECM, such as fibrin (Takahashi et al. 2022), laminin, hyaluronic acid, gelatin (i.e., hydrolyzed collagen), vitronectin, or ECM mixes such as Matrigel. Additional natural biomaterial examples include alginate (from algae; Scheffold 2022; Xiang et al. 2022), agarose and carrageenan (from seaweed, Marques et al. 2022), silk (from spiders, Johansson et al. 2018), chitosan (from crustaceans or fungi), cellulose (from many plants), konjac, glutenin (from wheat), zein (from corn), soy protein (Xiang et al. 2022), decellularized plant or animal tissues (discussed later), and fungal mycelium (chitosan, collagen, and cellulose reviewed in Campuzano and Pelling. 2020; Brodwin, 2018). A systematic comparison between a variety of non-animal-derived proteins and polysaccharide-based edible films revealed that protein-based films showed better cell adhesion properties and that patterned glutenin and zein films were better able to support cell alignment and differentiation compared to soy protein-based films (Xiang et al. 2022). These natural biomaterials are generally advantageous in that they have high biocompatibility, high degradability, and low immunogenicity; however, only biomaterials derived from vertebrate ECM contain native cell adhesion motifs.
While growing cells on biomaterials such as alginate or chitosan is possible, they lack recognition motifs that promote cell adhesion and migration, limiting their functionality. These cell recognition motifs include the Arg-Gly-Asp (RGD) and Ile-Lys-Val-Ala-Val (IKVAV, (Tashiro et al. 1989) motifs, amongst others less commonly used, derived from corresponding amino acid sequences in fibronectin and laminin, respectively (Bajaj et al. 2014). Integrins on the cell surface serve as the binding receptors for these motifs, mediating cell attachment and downstream signaling (Xiong et al. 2002). Many strategies exist that permit functionalization of biomaterials that lack recognition motifs, either by mixing with other functional biomaterials such as gelatin (Enrione et al. 2017), chemical crosslinking with RGD (Tsai et al. 2013) or laminin-derived peptides (Alheib et al. 2022), or genetically engineering the recognition motif directly into a biomaterial (Lee et al. 2016; Widhe et al. 2016). Functionalization of cellulose-based scaffolds can also be achieved using a cellulose binding domain-RGD fusion protein (Cohen 2022). As an alternative to functionalization with RGD or other recognition motifs, it was recently demonstrated that dextran could instead be functionalized with tyramine and then cross-linked to cell surface proteins using horseradish peroxidase (Kamperman et al. 2021). Surface functionalization methods such as ion beam deposition or plasma treatment can also be used to create favorable surface properties such as hydrophilicity (Rana et al. 2017).
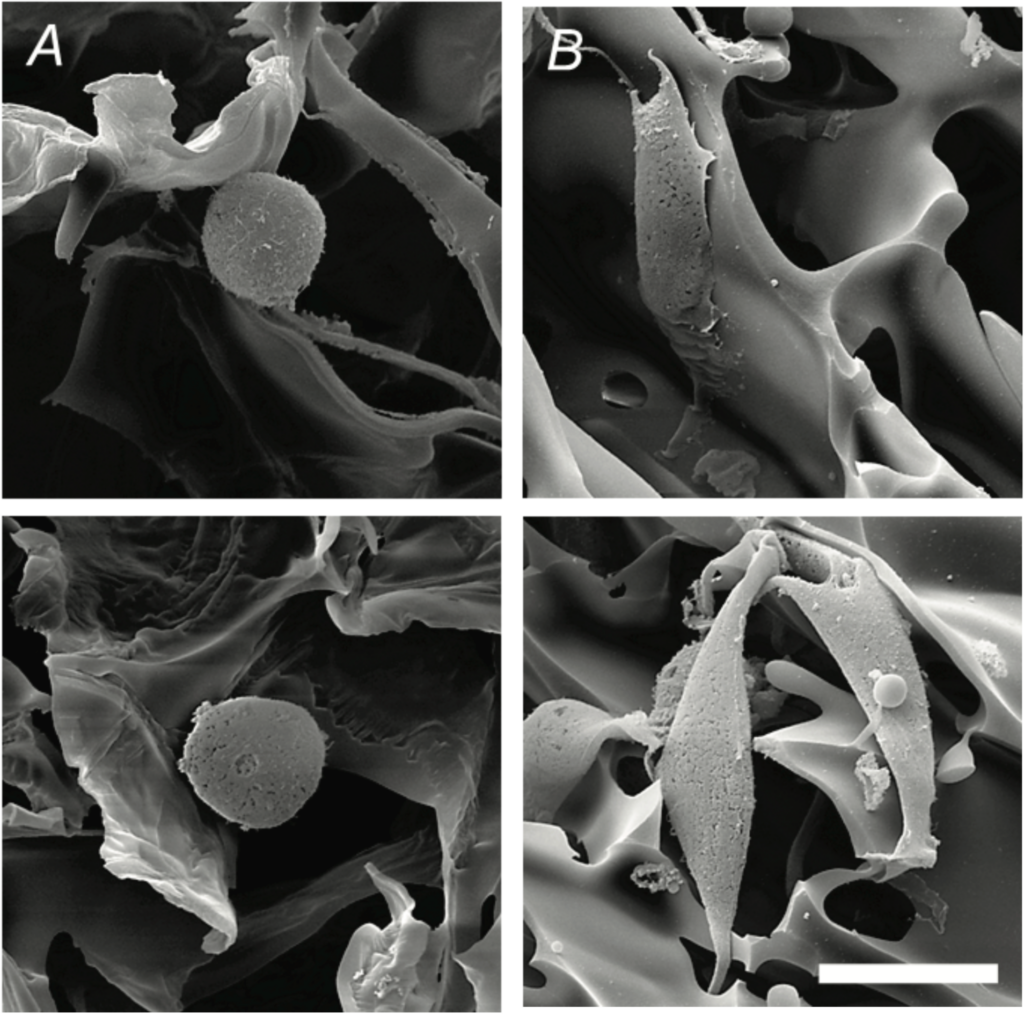
Alternatively, synthetic biomaterials can be used. Synthetic biomaterials permit high tunability for a variety of desired biophysical properties, making them attractive for tissue engineering purposes (Tibbitt and Anseth, 2009; Zhu and Marchant, 2011). Some common examples of synthetic biomaterials include Pluronic, poly(ethylene glycol) (PEG), polyglycolic acid (PGA), poly(2-hydroxy ethyl methacrylate) (PHEMA), and poly(acrylamide) (Rosales and Anseth, 2016). However, because these materials do not support cell adhesion, they also need to be functionalized as previously described, and are commonly combined with other natural polymers to form a composite material. Notably, RGD peptides have yet to be approved for use in food, which may hinder their use in cultivated meat applications.
Hydrogels
Although purified ECM proteins can be used to coat substrates for 2D cell growth, the use of a single protein component in 3D culture often results in a lack of desirable attributes such as mechanical properties, mesh network size, and degradation (Caliari and Burdick, 2016). Thus, a large effort has been dedicated to controlling these characteristics, discussed below, by creating composite hydrogels composed of synthetic and natural biomaterials.
The vast majority of applied biomaterial use in tissue engineering comes in the form of a hydrogel (Figure 6). A hydrogel is a 3D network of polymer chains that can readily absorb water (up to 1000x their dry weight) due to their hydrophilic properties. They can be engineered to swell or de-swell in response to various external stimuli (e.g. temperature, light, pH, electric field), and tuned for incorporation of a variety of macromolecules (Ahmed, 2015; Bajaj et al., 2014). Because of their aqueous makeup, hydrogels naturally have high permeability for oxygen and permit the flow of water-soluble molecules, exquisitely mimicking soft tissues of the body.
A hydrogel’s mechanical properties are frequently measured with atomic force microscopy to reveal the Young’s modulus value, which defines the material stiffness in relation to stress and strain. In vivo, the Young’s modulus of different tissue types is variable and this helps to determine cell fate via mechanotransduction. For instance, adipose tissue and the brain are soft (~0.2 – 1.0 kPa), skeletal muscle is intermediate (~10 kPa), and bone is hard (~30-45 kPa) tissue. Thus, recapitulating these stiffnesses within a scaffold can direct the differentiation of stem cells. For instance, the growth of mesenchymal stem cells on soft or hard hydrogels can bias their fate into fat or bone, respectively (Engler et al., 2006; Guvendiren and Burdick, 2012).
Similarly, muscle satellite cells can self-renew when grown in a substrate that matches the stiffness of their native stem cell niche (Gilbert et al. 2010; Safaee et al. 2017). Tuning of the stiffness can be achieved by increased crosslinking, addition of carbon nanotubes (Shin et al. 2012), graphene (Martín et al. 2017), DNA (Chen and Seelig, 2019a), or modification of natural or synthetic polymer materials with photo-crosslinkable side groups. Importantly, these photo-crosslinkable groups permit fast polymerization, allowing encapsulation of cells during a process such as bioprinting (discussed later). Cultivated meat scaffolds may thus be constructed with various composite polymer materials that dictate stiffness in a pre-patterned spatial orientation to replicate the fat, muscle, and connective tissue architecture found in a desired meat product. Indeed, studies have demonstrated the biasing of stem cell fates to bone and fat via stiffness properties within a single hydrogel (Freeman and Kelly, 2017). A recent study demonstrated that the stiffness of tyramine-functionalized dextran could be modulated during the course of the culture period, resulting in varying levels of adipogenesis and osteogenesis (Kamperman et al. 2021).
Alternatively, the hydrogel itself may be dissolved into a mixture of cell culture media and cells, assisting cell attachment and spreading. Mosa Meat describes using a cell-laden dissolved hydrogel to position cells in a dedicated apparatus for cell differentiation or structuring (Breemhaar and Post, 2019). Additionally, the properties of certain hydrogels may offer advantages when it comes to structuring end products. For example, Zagury et al. (2022) demonstrated that separate constructs consisting of alginate-based scaffolds loaded with differentiated muscle and fat cells could be combined into a single, cohesive construct by chelating calcium ions at the borders of the constructs, combining them, and then re-cross-linking them using calcium solution. This created a “marbled” construct without the need to identify conditions that allowed both cell types to efficiently differentiate under co-culture conditions.
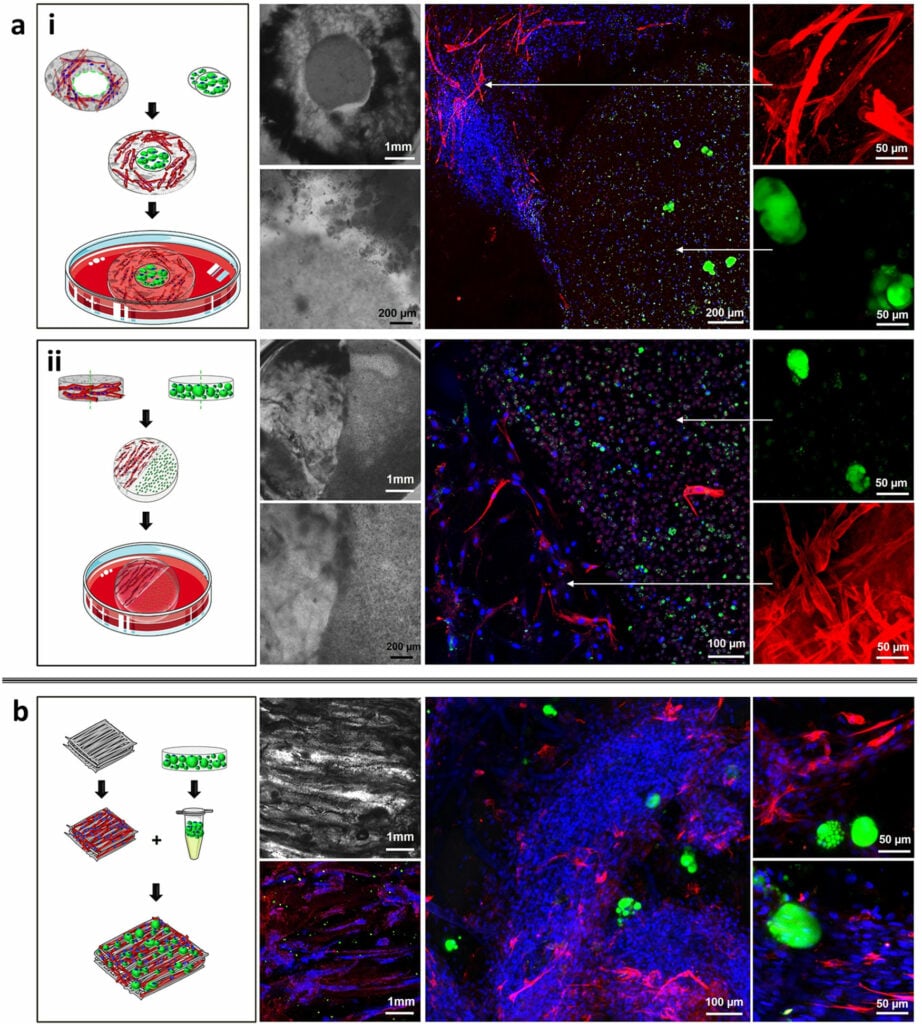
Although hydrogels can mimic soft tissues, they are limited in scale. Creating thick tissues that permit diffusion of oxygen and transport of nutrients and waste analogously to vascularized tissue in vivo has been challenging and is an active area of research. In general, a scaffold used for transplantation contains pores that permit neovascularization of the tissue once in the body (Bramfeldt et al. 2010). Scaffolds for cultivated meat will need to support cell viability via perfusion within a bioreactor (discussed later) rather than in situ vascularization. Creating vascular tissue from stem cells has been demonstrated at small scales but remains a challenge (Wimmer et al. 2019). For example, in the “cell-accumulation technique,” thin coatings of fibronectin and gelatin were used to facilitate adhesion between single cells, creating dense tissues capable of forming capillary networks when fibroblasts and endothelial cells were combined (Nishiguchi et al. 2011). Large 3D tissues can also be maintained in culture simply by creating pores in the scaffold that mimic the function of the vasculature without using vascular cells (Miller et al. 2012).
It remains to be seen whether the likely advantages of including endothelial cells in cultivated meat—their self-organizing properties and ability to prevent pores from closing up over time as the cell mass increases and the scaffold degrades—will outweigh the added difficulty of co-culturing an additional cell type. In a 2020 survey of cultivated meat manufacturers by GFI and TurtleTree Scientific, four out of nineteen companies reported plans to produce endothelial or other vascular cells within the next 12 months. Whether or not endothelial cells are used, a scaffold’s mesh network of pores should ideally be interconnected and distributed such that cells can infiltrate the scaffold and lie within 200µm of nutrient access, as this is the upper limit for the mass transfer of oxygen (Martin and Vermette, 2005). As an alternative to incorporating vascular-like networks in cultivated meat, large constructs may be created by stacking smaller hydrogel-based sheets after cells have already differentiated and matured (Furuhashi et al. 2021; Nie et al. 2021).
Other parameters such as pore shape, volume, and roughness also need to be considered (El-Sherbiny and Yacoub, 2013). Ideally, the recapitulation of the ECM should happen on the scale of the structural ECM components themselves (i.e. 50 – 500nm diameter, (Barnes et al., 2007), while the porosity of the scaffold should be on the micrometer scale to permit cell invasion and migration. This general principle has been challenging to mimic. However, sophisticated techniques for fabricating large hydrogel scaffolds with these properties are coming of age (discussed later). Lastly, given that a tissue created for consumption does not need to be functional inside of a body, a scaffold designed to be less densely populated and organized by its downstream sensorial properties may be easier to achieve. Once populated, the structure could be compressed at harvesting or further structured into a final product.
Careful design considerations have been made in tissue engineering to utilize non-immunogenic, biodegradable materials with biologically inert by-products (as they are intended to be inserted into the body for regenerative medicine purposes (Bajaj et al., 2014). Likewise, a scaffold that biodegrades into inert by-products would be desirable for cultivated meat not only to avoid non-edible materials being incorporated into a final product but also to allow the cells to replace the hydrogel scaffold with their own native ECM. Indeed, hydrogels are typically static substrates that alone fail to dynamically recapitulate the spatiotemporal interactions between a cell and the ECM. One method to overcome this is by incorporating proteolytically degradable crosslinks (Khetan et al., 2013; Patterson and Hubbell, 2010), which permit naturally secreted enzymes such as matrix metalloproteinases to degrade the hydrogel substrate, permitting cell migration and establishment of dynamic reciprocity previously described. A suite of other methods such as photodegradable polymers (Kloxin et al., 2009) and unique chemistries permitting light-mediated crosslinking (Guvendiren and Burdick, 2012) have also been developed by bioengineers, enabling more accurate recapitulation of ECM-cell dynamics.
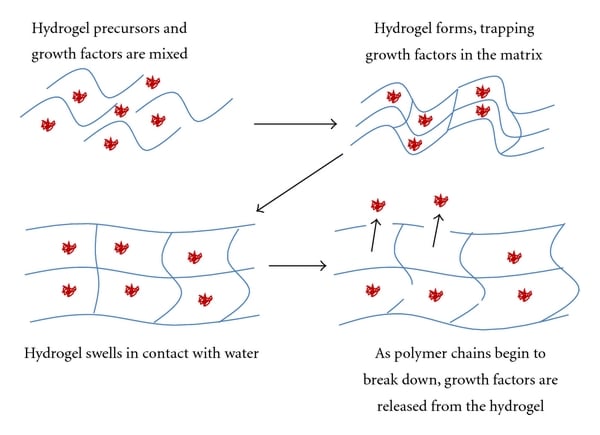
Bioengineers also attempt to mimic other characteristics of the ECM, such as regulated diffusion of soluble secreted signals (Figure 6). In vivo, ECM molecules such as heparan sulfate proteoglycans can bind and sequester growth factors that regulate local cell migration and differentiation. Some hydrogels, such as Matrigel, derived from mouse tumor cells, contain these embedded growth factors, albeit in variable quantities and unknown spatial distributions. Techniques such as incorporating proteins containing photocleavable linkers or photocaged peptides permit precise and sometimes reversible spatiotemporal release of sequestered factors via light (So et al. 2018). However, it’s unclear how feasible these methods would be for cultivated meat production at scale. Rather, covalent tethering of specific growth factors within the hydrogel scaffold (McCall et al. 2012), spatially distributed to mimic the cytoarchitecture of meat, may be able to assist in attachment or guidance of precursor stem cells into their correct location within the scaffold or toward downstream terminally differentiated cell fates. Indeed, related strategies have recently been demonstrated for cultivated meat. For example, chitosan and carboxymethylcellulose sheets were used as reservoirs for C-phycocyanin (C-PC), a seaweed-derived protein capable of partially substituting for the function of FBS, and a layer of agarose was used to modulate the rate of C-PC release (Park et al. 2021a). In a similar approach, gelatin microspheres (structurally similar to those used as microcarriers) supported the gradual release of C-PC and IGF-1 in reduced serum conditions, with positive effects on the properties of a small-scale C2C12-derived CM prototype (Park et al. 2021b). While both of these studies relied on scaffold-free cell sheets, the controlled release of high-value media components from hydrogels is applicable to both scaffold-based and scaffold-free strategies. GFI grantee Dr. Kelly Schultz is exploring the potential of hydrogel-based scaffolds to assist with muscle fiber alignment, nutrient delivery, waste removal, and scaleup.
How are scaffolds made?
The quest to build human organs from scratch has led to the development of an array of technologies and methods for reconstructing complex tissues. In general, two approaches can be considered for the biofabrication of a complex tissue: top-down and bottom-up. In top-down approaches, as generally described above, a scaffold is fabricated and cells are seeded onto the scaffold; however, these approaches generally struggle to recapitulate tissue microstructure (Nichol and Khademhosseini, 2009). Alternatively, bottom-up approaches aim to create modular biostructures on the microscale that can be assembled into larger, more complex tissue types. Advances in 3D printing now permit combinatorial approaches of both efforts (Daly and Kelly, 2019; Moroni et al., 2018). At the heart of each method is a concern for issues previously discussed: biomaterial selection, biocompatibility, porosity, etc. The emerging technologies that best handle these issues and those that are most likely to be explored for use in cultivated meat scaffold development—such as 3D bioprinting, polymer spinning, and decellularization—as well as other considerations, will be discussed in the next section.
Many natural polymers that can be used as scaffolds are also edible to humans, with several already approved by the FDA for use in food (regulation may differ by region). Thus, edible polymer materials in microcarriers or scaffolds for cultivated meat development are likely to be pursued in order to avoid removing cells from the scaffold or rigorous demonstration of safety for human consumption, as would be needed with some other material types. Research in this direction using edible scaffolds for cultivated meat has already begun. Examples of FDA-approved edible biomaterials include pectin (Munarin et al., 2012), gellan gum (Gong et al., 2009), chitosan, gelatin, cellulose, glucomannan, starch, gluten, and alginate, amongst others (Del Valle et al., 2017).
Recent demonstrations suggest that textured soy protein can serve as a viable scaffold for bovine stem cell attachment (>80% seeding efficiency without prior functionalization) and proliferation (Ben-Arye et al., 2020). Subsequent studies used coatings of natural polysaccharides (Lee et al. 2021 [article in Korean]) or a mixture of fish gelatin and agar (Lee et al. 2022) to further improve cell adhesion on textured soy protein scaffolds. The porous structure of bread has also been utilized as a scaffold (Holmes et al. 2021). Highly porous structures can also be created by freeze-drying hydrogels, a strategy that has been successfully applied in lab-scale cultivated meat prototypes (Li et al. 2022a). Furthermore, directional freezing can be used to create elongated and aligned pores (Jana et al. 2013; Rubio et al. 2019), which can be helpful for both promoting muscle cell differentiation and introducing large-scale texture or “grain” to the final cultivated meat product. Some synthetic polymers, such as PEGs, are also FDA-approved. As previously mentioned, the majority of these polymers lack functional domains for cell adhesion; however, they can be functionalized with RGD peptides or combined with functional edible components such as gelatin (Enrione et al. 2017). Importantly, these scaffolds also tend to be affordable and scalable in terms of the raw material components, with scale limitations mainly determined by the method of fabrication (discussed later). Some companies have independently demonstrated the large-scale production of proteins such as collagen using recombinant yeast or bacteria, and this may serve as a future platform for the creation of biomaterials used by the cultivated meat industry. Lastly, many of these biomaterials have also been used for tissue engineering of bone (Levengood and Zhang, 2014), which may be applicable for cultivated meat if bone-in products are ever pursued.
Scaffold-free methods
While the function of a scaffold is to mimic the properties of the ECM, it is also possible to avoid the use of scaffolds entirely by taking advantage of the fact that cells naturally secrete their own ECM material. For example, by stacking sheets of myogenic and/or adipogenic cells, researchers were able to create dense tissues (Shahin-Shamsabadi & Selvaganapathy, 2021; Tanaka et al. 2022). In a similar strategy, cells were cultured as spheroids and then aggregated into larger tissues using net molds (Sakaguchi et al. 2021). These methods have the advantage of producing high cell densities with naturally-occurring ECM proteins, the latter of which might be expected to lead to more conventional meat-like nutrition profiles and textural properties as well as avoiding regulatory hurdles associated with exogenous scaffold materials. However, scaling such methods may be difficult. Oxygen, nutrient, and waste diffusion limits the size of tissues that can be created. For tissues that need to be cultured after assembly, the lack of vascularization may be a serious challenge.
Organoids are small pieces of tissue that use the self-assembling properties inherent in pluripotent animal cells to reproduce features of in vivo tissue organization. Organoids can mimic the properties of a variety of tissues, including the somites from which skeletal muscle develops (Sanaki-Matsumiya et al. 2022). It may be possible to apply organoid technology to cultivated meat, an approach currently being pursued by Dr. Iftach Nachman’s team and Forsea Foods. At least in theory, organoids containing a functional vasculature could circumvent the size limitation associated with diffusion-limited constructs (Vargas-Valderrama et al. 2020). A major benefit of this methodology is the 3D growth microenvironment which permits a high degree of self-organization and maturation in relation to 2D growth (Hu et al. 2018). However, reproducibility in the relatively new organoid field is a challenge and an area of active research (Huch et al. 2017).
Additionally, transitioning cells from organoid growth onto a scaffold would be counterintuitive, as cells are already pre-patterned and self-organized. Thus, these methodologies may serve well for scaling cell numbers for direct use in unstructured products (discussed in End Products) but may not easily be incorporated into structured products containing prefabricated scaffolds. One possible alternative is the use of assembloids, in which two or more types of organoids containing different cell types are combined to form a spatially organized construct (Vogt, 2021). In the case of cultivated meat, muscle organoids could be combined with fat organoids to produce structured products. As mentioned above, similar strategies have already been demonstrated using muscle-laden and fat-laden microcarriers (Liu et al. 2022b).
It is noteworthy that UPSIDE Foods claims in documentation submitted to FDA (see page 26) that their product does not use or require any scaffolding material. This may be one piece of evidence in favor of the scalability potential of scaffold-free methods.
View references featured in scaffolding basics
Scaffold manufacturing technologies
Introduction
The range of meat products on the market exists on a spectrum of structural sophistication. On one end of the spectrum, there are less sophisticated processed products such as surimi and hot dogs; in the middle, minced products such as burgers and sausages; and on the other end, filets and steaks. In order to replicate the more sophisticated products, scientists will need to borrow and improve upon technologies from tissue engineering, regenerative medicine, and biomaterials science to recreate the complex multicellular architecture of meat. Building off of bioengineering and biomaterials knowledge discussed previously, a review of the core methods to consider for the creation of complex multicellular meat products are discussed below.
3D bioprinting
3D bioprinting is an additive manufacturing technique where pre-polymer solutions or pre-polymer solutions containing cells (i.e. a bioink) are deposited onto a substrate layer-by-layer under the guidance of a computer-aided design (CAD) process. The CAD files typically result from real bioimaging data such as magnetic resonance imaging (MRI) and computer tomography (CT) scans of tissues, but can also be user-generated to form limitless geometry types. Similar imaging strategies can be performed to replicate specific cuts of meat (Ebrahimnejad et al. 2018). Recently, 3D bioprinting has gained interest as a possible method for producing complex, structured cultivated meat products (Handral et al. 2020; Tibrewal et al. 2023). There are several types of bioprinting, summarized in (Bajaj et al. 2014; Derakhshanfar et al. 2018) and described in detail below.
Extrusion | Inkjet | Stereolithography | Laser-assisted | |
---|---|---|---|---|
Advantages | Simple, capable of printing various biomaterials, ability to print high cell densities | Ability to print low viscosity biomaterials, fast fabrication speed, low cost, high-resolution | Nozzle-free technique, printing time independent of complexity [1,2], high accuracy and cell viability | High resolution, deposition of biomaterials in solid or liquid phase |
Drawbacks | Only applicable for viscous liquids | Inherent inability to provide a continuous flow [3], poor functionality for vertical structures, low cell densities | UV light source and near-UV blue light’s toxicity to cells [4,5], lack of printing multi- cells, and damage to cells during photo curing [6] | High cost, thermal damage due to nanosecond/femtosecond laser irritation [7] |
Speed | Slow [8,9] | Fast [8,9] | Fast [9] | Medium [9] |
Cost | Moderate [3,10] | Low [3,10] | Low [3,10] | High [3,10] |
Vertical printing ability | Good [1] | Poor [1] | Good [1] | Medium [1] |
Cell viability | 89.46 ± 2.51% [11] | 80-95% [12, 13] | >90% [14,15] | <85% [7] |
Cell density | High [16] | Low [16] | Medium [16] | Medium [16] |
Resolution | 100 μm [3] | 50 μm [3] | 100 μm [14,15] | 100 μm [17] |
Viscosity | 30-6*107 mPa s [8] | <10 mPa s [8] | No limitation [2] | 1-300 mPa s [8] |
1: Wang et al. 2015b; 2: Mandrycky et al. 2016; 3: Ozbolat and Yu 2013; 4: Sinha and Häder 2002; 5: de Gruijl et al. 2001; 6: He et al. 2016; 7: Hopp et al. 2012; 8: Hölzl et al. 2016; 9: Xu et al. 2009; 10: Billiet et al. 2012; 11: Dai et al. 2017; 12: Saunders and Derby 2014; 13: Gao et al. 2015; 14: Gauvin et al. 2012; 15: Gou et al. 2014; 16: Murphy and Atala 2014; 17: Guillotin et al. 2013
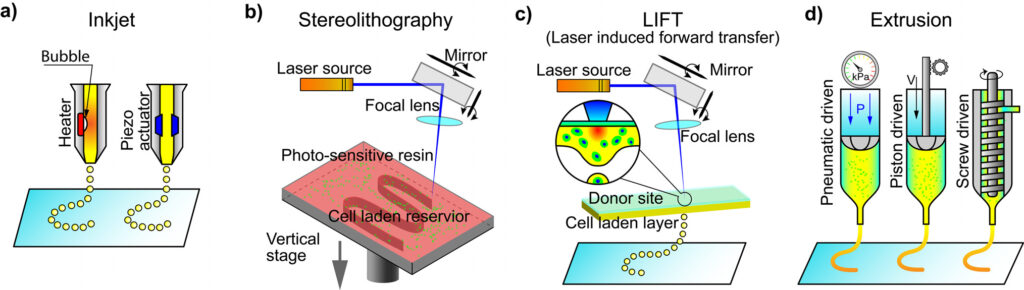
Bioink
Bioink is the raw material for 3D bioprinting, composed of cells in combination with some other biological material. There are two major types of bioink materials: scaffold-based and scaffold-free. A scaffold-based bioink is, in essence, a hydrogel that is printed along with cells whereas a scaffold-free bioink contains only large cellular components such as tissue strands or spheroids (Hospodiuk et al. 2017). Cells within a bioink typically exist at a concentration of ~10 million cells per milliliter, constituting ~5% or less of the bioink volume (Moroni et al. 2018a), although this can be optimized on a case-by-case basis to permit enough printed cells to replicate a specific tissue. The use of a two-phase emulsion bioink to print porous scaffolds was demonstrated to improve the proliferation and spreading of the seeded cells in comparison to a traditional single-phase bioink (Ying et al. 2018).
Importantly, however, not all biomaterials previously discussed fit the parameters for use in a bioink. The main parameters to consider for a bioink include the “bioprintability” or rheological properties (e.g. viscosity, thixotropy), mechanical properties, insolubility in cell culture medium, cost, and manufacturing capability. Because natural biomaterials generally have weak mechanical properties, special considerations need to be made when including them in a bioink. Additionally, each bioprinting method affects these parameters differently, thus the intended application tends to dictate the chosen method. For cultivated meat, a food-grade bioink would be necessary. Some examples of food-safe biomaterials that have been demonstrated in 3D bioprinting applications include zein (Tavares-Negrete et al. 2021), chitosan, whey protein (Yang et al. 2021), pea protein isolate, soy protein isolate, RGD-modified alginate (Ianovici et al. 2022; Zagury et al. 2022), photosensitive soybean oil-based resin (Sealy et al. 2022), κ-carrageenan (Marques et al. 2022), and a blend of gelatin, alginate, and protein hydrolysate (Dutta et al. 2022).
Extrusion bioprinting
In extrusion-based bioprinting, an extruder continuously deposits a bioink while the stage or the extruder itself is moved. Bioink deposition can occur via pneumatic or mechanical systems and the 2D ink must be rapidly solidified either chemically (e.g. light) or physically (e.g. temperature) prior to adding a new layer in order to maintain shape without spreading. In general, extrusion-based systems are the most used and versatile of the methods, permitting a wide variety of natural and synthetic biomaterials to be used due to the range (30 mPa•s to 60 x 10^6 mPa•s; for reference, cream (45% fat, 16 °C) has a viscosity of 48 mPa•s and lard or shortening (23 °C) has a viscosity of 1-2 x 10^6 mPa•s) of printable ink viscosities. Extrusion-based methods fundamentally work by dispensing larger filaments (~150-300µm in diameter) rather than droplets. This permits both scaffold-based or scaffold-free printing, capable of incorporating high cell density bioinks, printed spheroids, or cell-laden microcarriers (Hölzl et al. 2016).
However, this also results in poor printing resolution (as low as 100 µm) versus other methods, making intricate replication of complex tissues difficult, and there is often an inverse relationship between the achievable resolution and speed. Continuous chaotic bioprinting, in which a static mixer forms alternating layers of bioink and a spacer material (such as alginate), can partially address this problem by increasing the “effective” resolution beyond the actual width of the extruded fiber (Bolívar-Monsalve et al. 2021). Bioinks with shear thinning properties are preferable as shear forces naturally align hydrogel polymers for extrusion; however, careful consideration must be given to the shear force effect on cell viability during extrusion (Hospodiuk et al. 2017). Additionally, bioinks with low adhesion and surface tension are necessary to prevent nozzle clogging. 3D food printers that use extrusion-based bioprinting methods and the technologies (e.g. texture analyzers) used to analyze the food products may also be adaptable for cultivated meat. Finally, the bioprinting of plant-based proteins may be informative for the creation of cultivated meat scaffolds or replication of other mechanical, textural, or organoleptic properties of meat. These plant-based protein scaffolds could then be seeded with cultivated animal fat, resulting in a hybrid product. Off-the-shelf 3D bioprinted scaffold products can also be used in cultivated meat research and development.
Several recent advances show the potential for applying extrusion-based methods toward the creation of thick scaffolds or tissues applicable to cultivated meat production. For instance, researchers have developed hybrid bioprinting devices, where both vascular networks and cells are printed simultaneously under control of independent printing arms equipped with smart sensors to avoid collisions (Yu et al. 2014). This strategy permits continuous medium perfusion through the printed vascular networks while additional tissue is printed. Systems have also been developed where multiple bioinks can be extruded continuously with fast, dynamic switching between diverse bioinks, permitting printing of complex tissues composed of multiple cells and hydrogel materials (Liu et al. 2016; Skylar-Scott et al. 2019b).
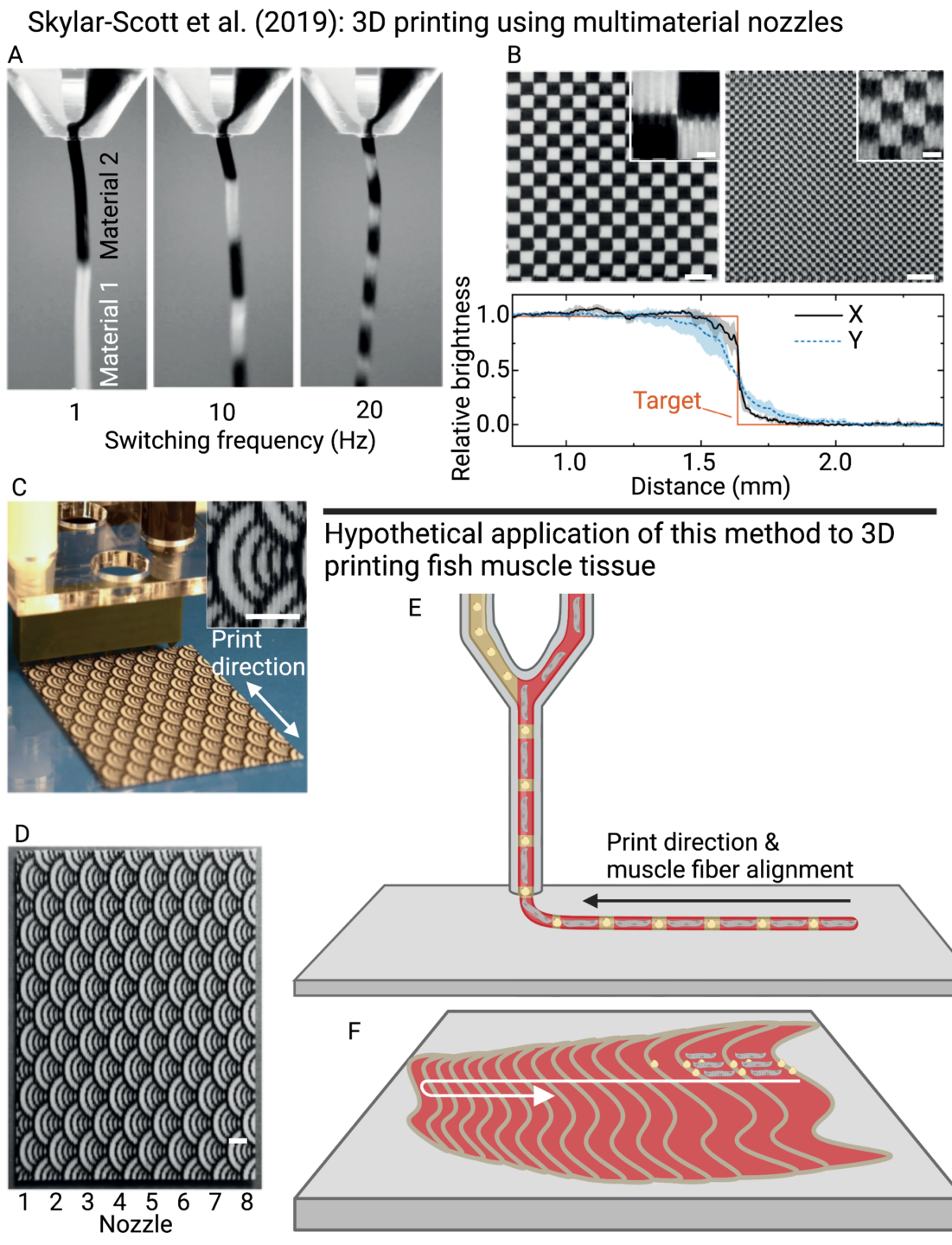
Another challenge in bioprinting thick scaffolds is the weak mechanical properties of natural polymers, resulting in their deformation under their own weight. To this end, the hydrogel can be printed within a second hydrogel that provides structural support (Video 1). The “sacrificial” support hydrogel acts as a Bingham plastic, where it is a solid up until the point of contact with the extrusion nozzle, permitting the printed hydrogel to displace the support hydrogel (Hinton et al. 2015). The support hydrogel can then be removed (i.e. dissolved) by increasing the temperature (e.g. when using gelatin), or applying calcium chelators (e.g. EDTA when using alginate) or enzymes, resulting in a standalone scaffold or cell-laden tissue. A recently-reported variation of this strategy referred to as “CarGrow” involved printing into κ-carrageenan microgels and then, rather than removing the support hydrogel, using it to support the construct during culture (Machour et al. 2022). The presence of the support hydrogel during the culture period prevented construct shrinkage and led to higher cell viability, which the authors suggested might be related to the lower cell density (as a result of the larger construct volume), improved ability to exchange nutrients and waste, and altered mechanical cues. Importantly, the prevention of construct shrinkage lasted only as long as the support hydrogel was in place, and removal of the gel following culture still allowed shrinkage to occur. This is likely to be an advantage for cultivated meat, allowing the cells to flourish within a less constrained environment but still producing a dense piece of tissue as a final product. Sacrificial gels have also been adopted for the creation of artificial vasculature (Ji et al. 2019). Additionally, the benefits of these aforementioned methods can be combined, enabling bioprinting of human-scale tissues with fewer limitations (Kang et al. 2016).
Lastly, as described previously, the spatiotemporal release of biomolecules within a scaffold can assist in biomimicry of the ECM. To this end, 3D-printed light-sensitive nanocapsules containing growth factors can be dispersed within a scaffold during printing, permitting guided migration of cells in a spatiotemporally controlled fashion via light (Gupta et al. 2015; Meng et al. 2019). These and other advances in extrusion bioprinting hold great promise for their application to the cultivated meat industry. Several companies have plans to utilize extrusion-based bioprinting in their cultivated meat production pipeline.
These and other advances in extrusion bioprinting hold great promise for their application to the cultivated meat industry. For example, a method referred to as tendon-gel integrated bioprinting in which collagen ink was used to produce artificial “tendons” as anchors at either end of the construct was used to produce a small steak-like prototype (Kang et al. 2021). A bioink consisting of gelatin-methacrylate, silk fibroin, and porcine satellite cells was used to print grid-shaped constructs capable of supporting cell survival and proliferation (Li et al. 2021). Printing speed and extrusion pressure have also been optimized for alginate and alginate+gelatin bioinks for the purposes of cultivated meat (Zanderigo 2022) 3D bioprinting has also recently been applied for the creation of cultivated pork fat (Song et al. 2022). In another recent study, large yellow croaker cells were printed using a bioink consisting of fish gelatin and alginate (Xu et al. 2023). A research project led by GFI grantee Dr. Frederico Ferreira aims to combine extrusion bioprinting and electrospinning (discussed below) to produce whole-cut cultivated fish with aligned myofibers (Marques et al. 2022), and one led by Dr. Sara Oliveira uses edible polymers to improve the texture and nutrition profile of cultivated meat. Several companies have plans to utilize extrusion-based bioprinting in their cultivated meat production pipeline.
Stereolithography
Stereolithography uses single or multiphoton light sources to rapidly polymerize a bath of light-sensitive pre-polymers. Structures derived from CAD models are recreated via light reflecting off of micromirror galvanometers, which provide feedback to the printing apparatus, enabling precise spatial and temporal control of the polymerization. Polymerization occurs either through direct laser light exposure or exposure through a mask in a bottom-up process of sequential 2D layers (Raman and Bashir 2015). Inclusion of photocrosslinkable biomaterials previously described now permits this technique to be used for scaffold generation or printing of structures from cell-laden pre-polymer baths. The advantages of stereolithography include avoidance of previously described considerations (e.g. viscosity, surface tension, and shear stress through a nozzle), automated control (in maskless methods), high resolution (< 100 µm), and fast printing speed. Disadvantages include potential cytotoxicity from light exposure and a limited but growing repertoire of suitable biomaterials to use. Further developments are also necessary to permit further scaling to more complex 3D constructs as well as derivation of food-safe and non-cytotoxic photoinitiators that assist in the crosslinking reaction during polymerization (Raman and Bashir 2015). Importantly, because photocrosslinking is also used to strengthen edible films in the food industry, the development of safe and edible photocrosslinkers is already an active area of research (Li et al. 2020).
Recent progress demonstrates the potential for using stereolithography in cultivated meat scaffold production. For instance, combining stereolithography with dielectrophoresis can enable precise spatial control of cells (Bajaj et al. 2013) and advances in microscale continuous projection printing enables sub-10µm resolution objects to be printed on the order of seconds (Soman et al. 2013). A related technique, digital light processing (DLP) printing, was recently demonstrated as a method for producing a small-scale cultivated steak prototype from bovine myogenic and adipogenic cells (Jeong et al. 2022). A variation on DLP in which the collector plate is replaced by a rotating cylindrical collector has been proposed as a possible method for improving the scalability of cultivated meat while allowing for tunable stiffness of the hydrogel (Garrett et al. 2021; Garrett 2022) A soybean oil-based resin has also been investigated as a potential photosensitive material for use in cultivated meat scaffolding (Sealy et al. 2022). Computed axial lithography uses angled image projections that result in concurrent 3D printing of whole structures at high speeds (Kelly et al. 2019). As a non-layered approach, it does not require the use of supportive hydrogels (discussed previously) for mechanically weaker biomaterials and should be readily scalable. Stereolithographic techniques have also recently demonstrated the construction of intricate vasculature using common, photo-absorbable food dyes such as tartrazine and anthocyanin (Grigoryan et al. 2019). Thus, further development of stereolithographic techniques may permit their use in the cultivated meat industry.
Droplet-based bioprinting
In droplet-based bioprinting, picoliter droplets (10-50µm diameter) are deposited on top of a substrate without direct contact being made between the nozzle and the substrate. Inkjet printing is the most common form of droplet-based bioprinting and can be further divided into whether droplets are created via electric, acoustic, or heat sources (Hospodiuk et al. 2017). Of these, piezoelectric and thermal methods are frequently used, where an electric charge or sharp increase in heat produces pressure increases at the nozzle, forming droplets. Droplet-based methods have the advantage of precise control of cell numbers distributed in each droplet and high resolution printing (10-50 µm), however, the applications for droplet-based printing of complex structures is diminished due to a low range of bioink viscosities (3.5 – 12 mPa•s) and requirements of low surface tension and rare rheopectic traits (Hospodiuk et al. 2017). For these reasons, it’s unlikely that droplet-based bioprinting would be utilized for cultivated meat.
Laser-assisted bioprinting
Laser-assisted bioprinting, also commonly referred to as laser-induced forward transfer (LIFT), uses laser-induced energy transfer to control deposition of bioinks. The LIFT system features an absorbing donor layer comprised of gold or titanium and a bioink layer beneath the donor layer. A focused laser beam vaporizes the donor layer, creating a high-pressure bubble that deposits the bioink to a substrate underneath, where it is subsequently crosslinked (Mandrycky et al. 2016; Moroni et al. 2018). Laser-assisted methods are able to avoid problems with mechanical stresses and are capable of bioprinting viscous bioinks (1 – 300 mPa•s) with high cell viability and high resolution (10 – 100µm, Hölzl et al. 2016). However, the apparatus cost is high and scalability is an issue. For these reasons, laser-assisted methods are less likely to translate to the cultivated meat industry.
Electrospinning and other spun fiber production methods
Electrospinning is a versatile, low-cost technique where a solution of polymers is passed through a spinneret needle during application of a high electric field. The electric field causes stretching of the polymer via electrostatic forces (Video 2) until a critical point (i.e. Taylor cone) is reached, resulting in the formation of a fiber jet. As the fiber jet is formed, the solvent evaporates off, and the fiber hardens while it is harvested on an electrostatically-grounded collection device (Video 3, Wang et al. 2013). The fiber itself can be made from a variety of synthetic and natural polymers, resulting in porous fiber networks with tunable diameters and high surface area. Electrospinning is unique in that it provides the only way to achieve nanoscale biomimicry of the ECM (50 – 500 nm diameter fibers). Of note, cell-laden biomaterials can also be electrosprayed through a similar process that yields droplet deposition rather than fiber deposition (Weidenbacher et al. 2017), although this is less applicable toward scaffold creation. Lastly, similar fiber production methods are possible such as wet, dry, or melt spinning, and solution blow spinning (Daristotle et al. 2016) that can be used to produce materials such as scaffolds (Farrugia et al. 2013) and hollow fiber membranes (Li et al. 1994) used in bioreactors (discussed in Bioprocess Design). Whereas electrospinning is usually carried out using direct current, alternating current electrospinning may present some scalability advantages and does not require a grounded collector. Alternating current electrospinning is being commercialized by the company Truspin.
One form of wet spinning is immersion rotary jet spinning (Gonzalez et al. 2016), which uses centrifugal force to extrude polymers into a liquid precipitation bath. This technique can produce fibers of various diameters at two to four orders of magnitude higher rates versus comparable electrospinning systems and can be used with a broader range of polymer materials (Figure 9). Immersion rotary jet spinning has been used to create edible gelatin fiber sheets that can be seeded with cells, demonstrating that some textural aspects of conventional meat can be replicated using this technique (MacQueen et al. 2019). However, the cell-laden gelatin sheets that were used were thin (1.5 mm thickness) and did not contain differentiated skeletal muscle cells or adipocytes. Thus, further work needs to be done exploring how these variables affect textural properties.
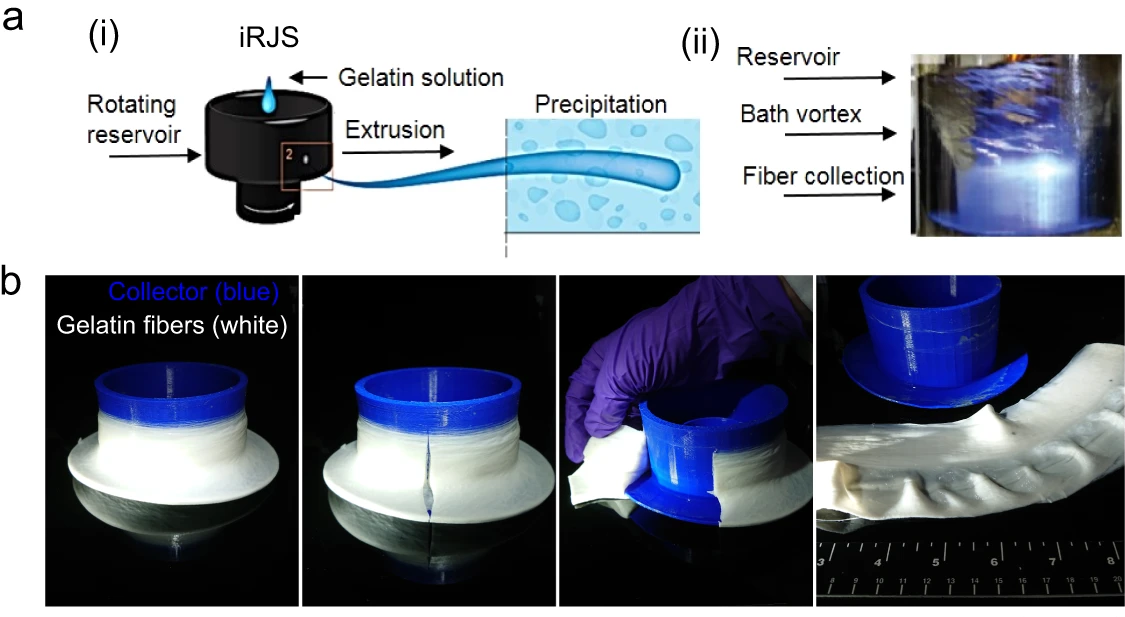
Many of the same considerations and principles previously discussed for biomaterials in hydrogel formation, bioinks, and bioprinting also apply to the creation of electrospun scaffolds. For instance, mechanically weak but biocompatible natural polymers are often combined with synthetic polymers. For example, a combination of polycaprolactone (PCL) and gelatin or collagen was used to produce both random and aligned electrospun scaffolds capable of supporting the growth of L6 rat skeletal myoblasts (Perez-Puyana et al. 2021). Identifying rapidly evaporating solvents suitable for composite materials can be challenging, however, and the solvents themselves may be cytotoxic (Cheng et al. 2017) or damage the native structure of the polymers (Bürck et al. 2013). Similarly to a bioink, alterations in viscosity, solvent surface tension, and flow rate can affect the fiber characteristics, and alterations in the applied voltage and rotating speed, distance from, or structure of the collection device can affect resultant scaffold geometries (Cheng et al. 2017; Khorshidi et al. 2016). Unless otherwise intended, electrospun scaffolds will result in a random arrangement of packed fibers, which can limit cell infiltration, migration, or uniform seeding (Khorshidi et al. 2016). Similar methods used for hydrogels, such as inclusion of protease-degradable polymers can assist in better mimicking native ECM for cell infiltration and migration (Wade et al. 2015). Additionally, a variety of methods have been developed in order to produce electrospun scaffolds with defined structure and purpose, including mixed, multilayer, coaxial, and emulsion electrospinning, and microfluidic spinning, discussed below.
While true biomimicry of the ECM requires nanoscale fibers, the scaffold’s porosity itself should be on the microscale to permit cell infiltration and perfusion. A fiber network on both nano and microscales can be created via multilayer electrospinning, where different nanofiber and microfiber polymers are electrospun sequentially in layers. Alternatively, mixed electrospinning can be performed where nanofiber and microfiber polymer types are simultaneously electrospun on a collector that permits overlapping collection through the lateral movement of the collector (Kidoaki et al. 2005). These techniques can permit or restrict certain cells from infiltrating a scaffold based on pore size (Ju et al. 2010), which may be leveraged for the spatial distribution of cell seeding for cultivated meat. Sacrificial biomaterials such as gelatin can also be used as a polymer to create additional pores upon melting.
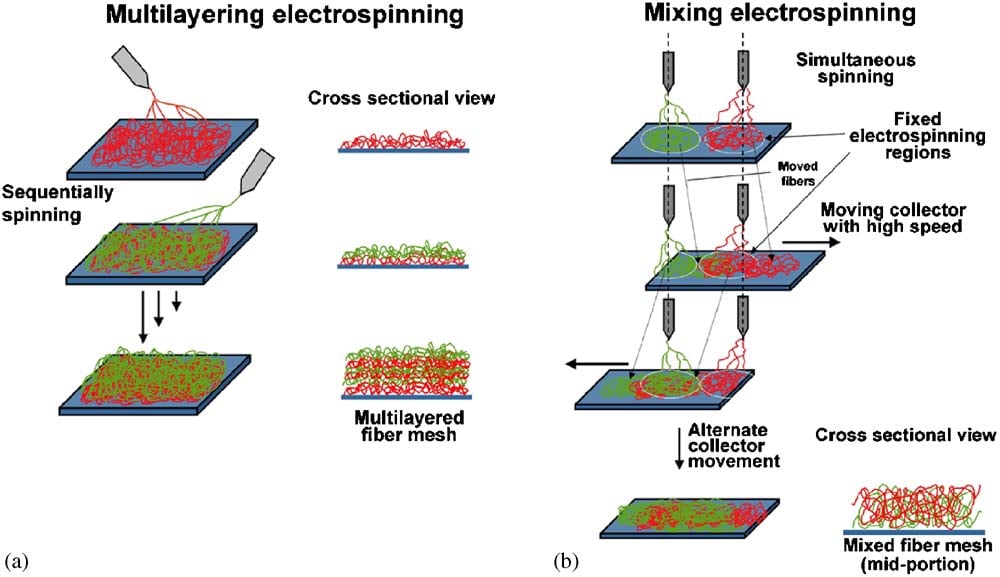
In coaxial electrospinning, an inner and outer solution is co-electrospun via independent feeding capillaries, forming a compound Taylor cone. One solution typically contains a hydrophobic polymer and the other contains a biological cargo of interest solubilized with hydrophilic polymers (e.g. dextran), resulting in a core-shell structure due to their immiscibility, or inability to mix (Rim et al. 2013). Coaxial electrospun fibers permit trapping of proteins, cells (discussed later), or other biomolecules such that their release kinetics can be varied based on the porosity or biodegradability of the outer shell polymer (Ji et al. 2010), which may serve well for embedding of growth factors for cultivated meat applications. The viscosity of the solutions, flow rate, and applied electric field strength can all influence the geometry and characteristics of the resultant core-shell fibers. Additionally, multi-layered fibers can be created by co-electrospinning more than 2 solutions (Labbaf et al. 2014). Similar core-shell fibers can be created via emulsion electrospinning where an emulsion of oil, water, surfactant, and biomaterial are co-electrospun. During electrospinning, the droplets in the emulsion coalesce due to increased viscosity of the evaporating oil and applied electric field. This process can produce core-shell fibers that can be embedded with growth factors or drugs, which may be leveraged for cultivated meat scaffolding (Nikmaram et al. 2017).
Microfluidic spinning relies on differences in a fluid’s surface tension, energy dissipation, and fluidic resistance to produce a 3D coaxial flow between independent sample and sheath flows (Cheng et al. 2017). While similar in principle to coaxial electrospinning, the application of voltage is unnecessary, as the fluidic channel width controls fiber diameter. Thus, microfluidic spinning permits a more favorable environment for natural biomaterials due to the avoidance of harsh solvents. In contrast to electrospinning, microfluidic spinning can more easily achieve uniformity of fiber diameter, as well as tighter control over the shape, porosity, and diameter of the fiber. This allows for simple cell encapsulation, which can assist in cell alignment, proliferation, and growth in a 3D environment, all of which are favorable for cultivated meat production (discussed later). The resultant fibers must be solidified via photopolymerization, crosslinking, or solvent exchange, which somewhat limits the selection of polymers. Nevertheless, easily crosslinked materials such as alginate can be readily used in such a system.
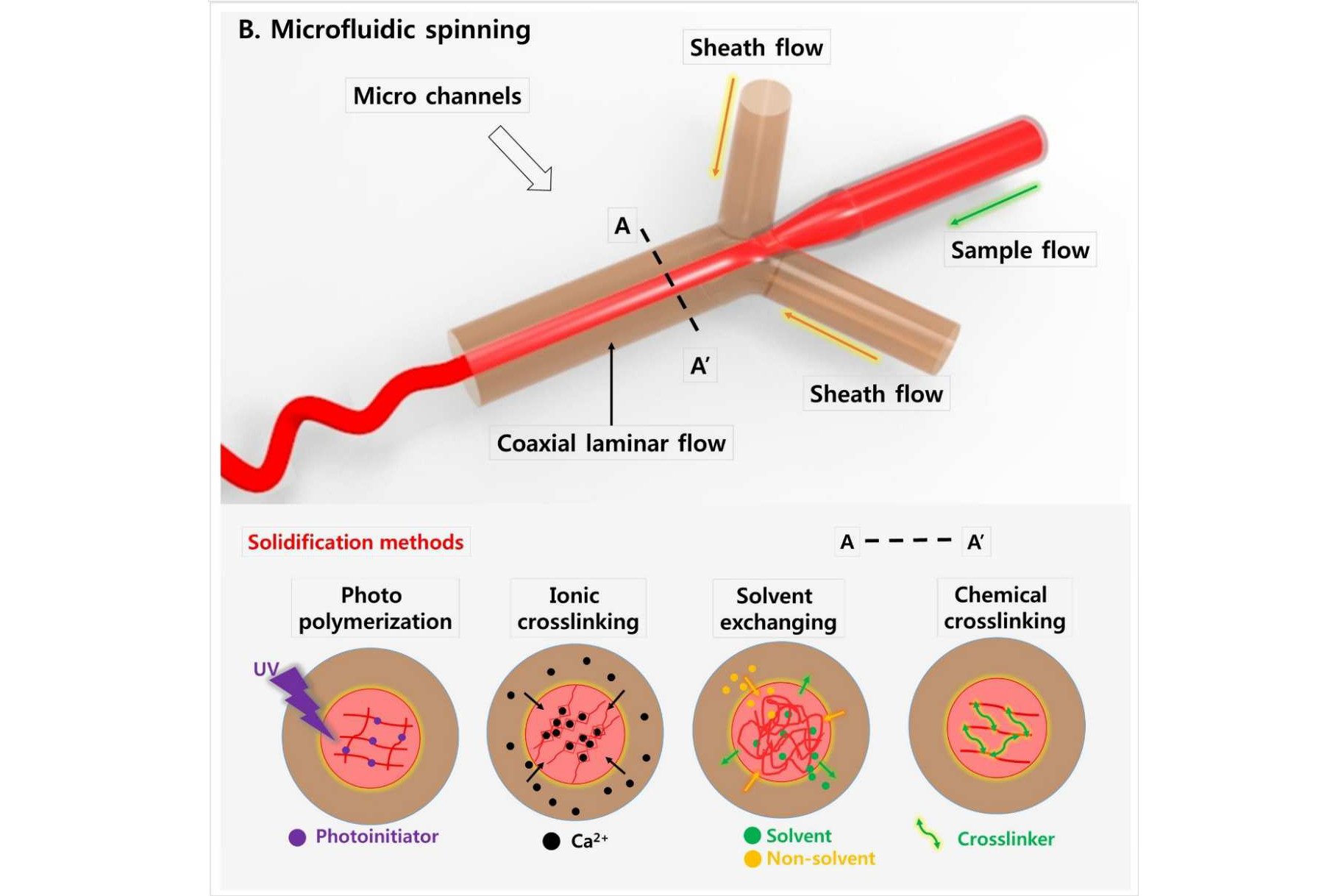
A similar method for creating nano- and microscale fibers is gravity fiber drawing, which relies on the tendency of a falling droplet with the correct composition to create a “tail” that solidifies into a fiber as the solvent evaporates (Yadavalli et al. 2020). This method offers precise control over fiber diameter and spacing and is being explored by researchers at the University of Georgia and the startup Cytonest for use as scaffolds for cultivated shrimp, supported by a grant from GFI.
Decellularization
Decellularization is a technique that removes the cells and nucleic acids from a tissue while preserving the native ECM. Thus, the decellularized tissue acts as a scaffold with preserved ultrastructure and similar biophysical and biochemical properties of the original tissue, potentially avoiding previously discussed issues (e.g. porosity, material composition, stiffness, etc) associated with bottom-up approaches. Decellularization has been largely pioneered using animal tissues for the purposes of organ regeneration, however, the technique can also be applied to plant or fungal tissues (Harris et al. 2021). As such, it is likely that the knowledge and application of techniques originating from animal tissues could be useful for cultivated meat research and development purposes, while real-world implementation is more likely to utilize plant or fungal tissues (Lu et al. 2022).
Decellularization is performed by deploying chemical, physical, or enzymatic methods. Chemicals such as ionic (e.g. SDS), nonionic (e.g Triton X-100), or zwitterionic (e.g. CHAPS) detergents can be used as they assist in solubilizing membranes, disrupting DNA-protein and lipid-protein interactions, or protecting a protein’s native state, respectively (White et al. 2017). However, the harsh nature of these chemicals leads to their use in low amounts or not at all, if possible, as they inherently will also damage ECM proteins (Badylak et al. 2011). In some cases, the use of acids to catalyze hydrolytic degradation of macromolecules, or organic solvents and hypotonic solutions to cause cell lysis can assist in decellularization processes. Physical methods such as freezing and thawing, electroporation, agitation, and sonication can also be applied to assist in cell lysis. Lastly, various enzymes can assist in the decellularization process, including trypsin, dispase, phospholipases, exonucleases, endonucleases, and other proteases, although consideration has to again be paid to balancing the damage done to ECM proteins (Gupta et al. 2018). As different tissues consist of different cell compositions, sizes, and densities, each decellularization protocol needs to be optimized on a case-by-case basis, and may include one or combinations of the methods described above.
In general, the decellularization process is a balance between removing cells and nucleic acids and maintaining the integrity of the native ECM. Near-complete removal of these components is critical in order to avoid immune responses from the host in the context of organ transplantation. However, this is less of a concern for cultivated meat as the tissue is dead and ingested rather than intended to survive in a host. Interestingly, some evidence suggests that residual antimicrobial peptides persist in some animal ECM scaffolds as well as chitosan (Kong et al. 2010), which may instead be leveraged to prevent contamination in vitro or following product packaging (Brennan et al. 2006). In summary, the cultivated meat field can learn from and apply the knowledge gained from decellularization and recellularization processes, discussed below.
The most applicable decellularization techniques for cultivated meat are perfusion and immersion with agitation. Perfusion is typically used for whole organ decellularization, where native vasculature (typically the main organ artery) is co-opted and used as the plumbing for perfusion of decellularization reagents at physiological pressures. This is often performed in a perfusion bioreactor (discussed in Bioprocess Design) where the physiological pressure ensures that the tissue is not damaged and that the decellularization reagents can effectively permeate all of the preserved vasculature, including capillaries. For tissues without accessible or large vasculature routes (e.g. skeletal muscle, skin), the tissue can be immersed in decellularization reagents and agitated, assisting in permeation of the tissue via diffusion (Garreta et al. 2017). While immersion methods do not require the need for a specialized bioreactor, they often can result in long exposures to the decellularization reagents and intracellular release of native proteases that can damage the ECM scaffold (Gupta et al. 2018).
Decellularization has come of age since the first demonstration of a decellularized rat heart in 2008 (Ott et al. 2008). Since then, decellularization of large, complex tissues such as human hearts (Guyette et al. 2016) and limbs (Gerli et al. 2018) has been achieved, as well as decellularization of cultivated meat-relevant tissue types such as whole muscles (Zhang et al. 2016). Information on the decellularized scaffold can be gleaned from microCT, scanning electron microscopy, atomic force microscopy, and immunohistochemistry, which yields information about the 3D architecture, biomechanical properties, and composition of the ECM, respectively (Garreta et al. 2017). This information can be leveraged for the recreation of scaffolds for cultivated meat, particularly through characterization of decellularized skeletal muscle (Wolf et al. 2012) and specific cuts of meat. Additionally, the information may be used in bottom-up scaffold approaches such as the creation of CAD models for 3D bioprinting or the solubilization of the native decellularized ECM itself for use in bioinks (Pati et al. 2014) or electrospinning (Young et al. 2017).
While decellularization has become relatively straightforward, a recellularization process may require additional optimizations in cell delivery and bioreactor engineering. For recellularization, cells are typically delivered via the vascular perfusion line at or below physiological flow rates over multiple infusions. Physiological flow rates assist in preventing structural damage to the ECM and protecting cells from shear stress, and these techniques have resulted in seeding efficiencies of organ parenchyma above 90% (Bijonowski et al. 2013). Pre-coating the scaffold with biomaterials such as chitosan, collagen, gelatin, or RGD peptides can additionally assist in increasing reseeding efficiency (Gupta et al. 2018). As a tissue is recellularized, flow pressure may need to be dynamically adjusted as porosity becomes limited (due to cell attachment), and monitoring systems may need to be developed for this purpose (Lawrence et al. 2009). Additionally, establishing endothelial cells and vasculature may require pulsatile flow to more accurately mimic the experienced physiological environment of these cell types. However, it’s uncertain if scaffold endothelialization would be necessary for cultivated meat production (Badylak et al. 2011). At least one company in the field has cited the creation of vasculature for their products. Bovine endothelial and smooth muscle cells enhanced myogenesis and ECM deposition of bovine myoblasts when grown on a textured soy protein scaffold, though under the conditions tested, this effect was mainly attributable to the smooth muscle cells rather than the endothelial cells (Ben-Arye et al. 2020).
One of the advantages of using a decellularized animal tissue as a scaffold is that it retains the “zip code” locations of the native cytoarchitecture encoded via residual embedded growth factors, mechanical properties, and other ECM characteristics previously discussed. Therefore, the process of recellularization is highly self-organized but still commands consideration for a cell population’s differentiation state prior to recellularization. All of the starting cell types desirable for cultivated meat production (discussed in Cell Lines) have been utilized for recellularization, including myoblasts (Jank et al. 2015), mesenchymal stem cells (Chandrika et al. 2019), embryonic stem cells (Nakayama et al. 2013), and induced pluripotent stem cells (Jaramillo et al. 2018). Indeed, similarly to experiments on hydrogel scaffolds, evidence suggests that recellularization of a decellularized animal tissue scaffold with pluripotent stem cells is sufficient to direct the differentiation toward the native tissue type, increasing expression of relevant cell type markers and functionality versus 2D protocols (Jaramillo et al. 2018), as well as assemble native substructures due to local microenvironments (Nakayama et al. 2013).
Likewise, recellularization with multipotent progenitors such as mesenchymal stem cells or induced pluripotent stem cell-derived progenitors can be directed into functional tissues made up of a variety of differentiated cell types (Chandrika et al. 2019; Kitano et al. 2017). Successful recellularization (and subsequent functional transplantation) has been performed on tissues as large as adult pig lungs in perfusion bioreactors up to dozens of liters in volume, suggesting that the process is scalable (Nichols et al. 2018). Thus, adoption of learned principles from recellularization or recreation of a decellularized scaffold via methods such as 3D printing with solubilized native ECM (Choi et al. 2016; Choi et al. 2019) should be highly applicable for cultivated meat research and development.
Use of decellularized scaffolds from animals has several limitations. For instance, the composition of a decellularized scaffold can vary between individuals and be affected by age and protocol irreproducibility (Gershlak et al. 2017). Most importantly, however, the supply is limited and thus expensive, and reconstructing an ECM containing up to hundreds of proteins is not feasible (Nakayama et al. 2013). For these reasons, plants have been an attractive alternative for decellularization strategies, as their cell walls are composed of abundant biocompatible polysaccharides such as cellulose, pectin, and hemicellulose, and native vasculature similar to animals that follows Murray’s Law, allowing perfusion. Indeed, in combination with functionalization methods previously discussed, attachment of mammalian cells onto decellularized plant scaffolds has been demonstrated using a variety of plant stems, leaves, or hypanthium tissues (Fontana et al. 2017; Modulevsky et al. 2014). Functionalized plant scaffolds have demonstrated long-term viability and cell proliferation, although differences in native stiffness, hydrophilicity, topography, and pore sizes can influence their success (Fontana et al. 2017). Future genetic engineering efforts could modify plants to express biocompatible protein domains (e.g. RGD), as done in silk (Widhe et al. 2016).
The variety of native tissue architectures of plants can be leveraged to recapitulate different meat cuts. For instance, decellularized celery (Campuzano et al. 2020), green onion (Cheng et al. 2020), and grass (Allan et al. 2021) have been demonstrated to create aligned myofibers. Aligned growth of fibroblasts has also been demonstrated on decellularized seaweed, suggesting that this might be another viable candidate for skeletal muscle tissue engineering, including cultivated meat (Bar-Shai et al. 2021). In part due to their prominent vascular networks, spinach leaves were investigated as scaffolds for cultivated meat, and it was shown that they were capable of supporting the growth and alignment of primary bovine satellite cells (Jones et al. 2021). Decellularized jackfruit produced colors and patterns similar to marbled meat. When tested as a scaffold for porcine myoblasts, this material was found to support cell adhesion when coated with fibronectin (Ong et al. 2021). Importantly, plant scaffold construction can be readily scaled simply by using larger or more abundant plant tissues, or processing of raw plant materials to create textured protein- or cellulose-based scaffolds using previously discussed methods (Krona et al. 2017). Recently, decellularized broccoli florets were shown to be effective as microcarriers for primary bovine satellite cells (Thyden et al. 2022). Plant-based materials will likely be significantly more cost-efficient at scale versus their animal ECM counterparts, as the processing and use of abundant raw plant materials such as cellulose or starches for tissue engineering are well characterized (Jovic et al. 2019) and recombinant technologies for animal proteins are likely to remain more expensive due to more inputs for production.
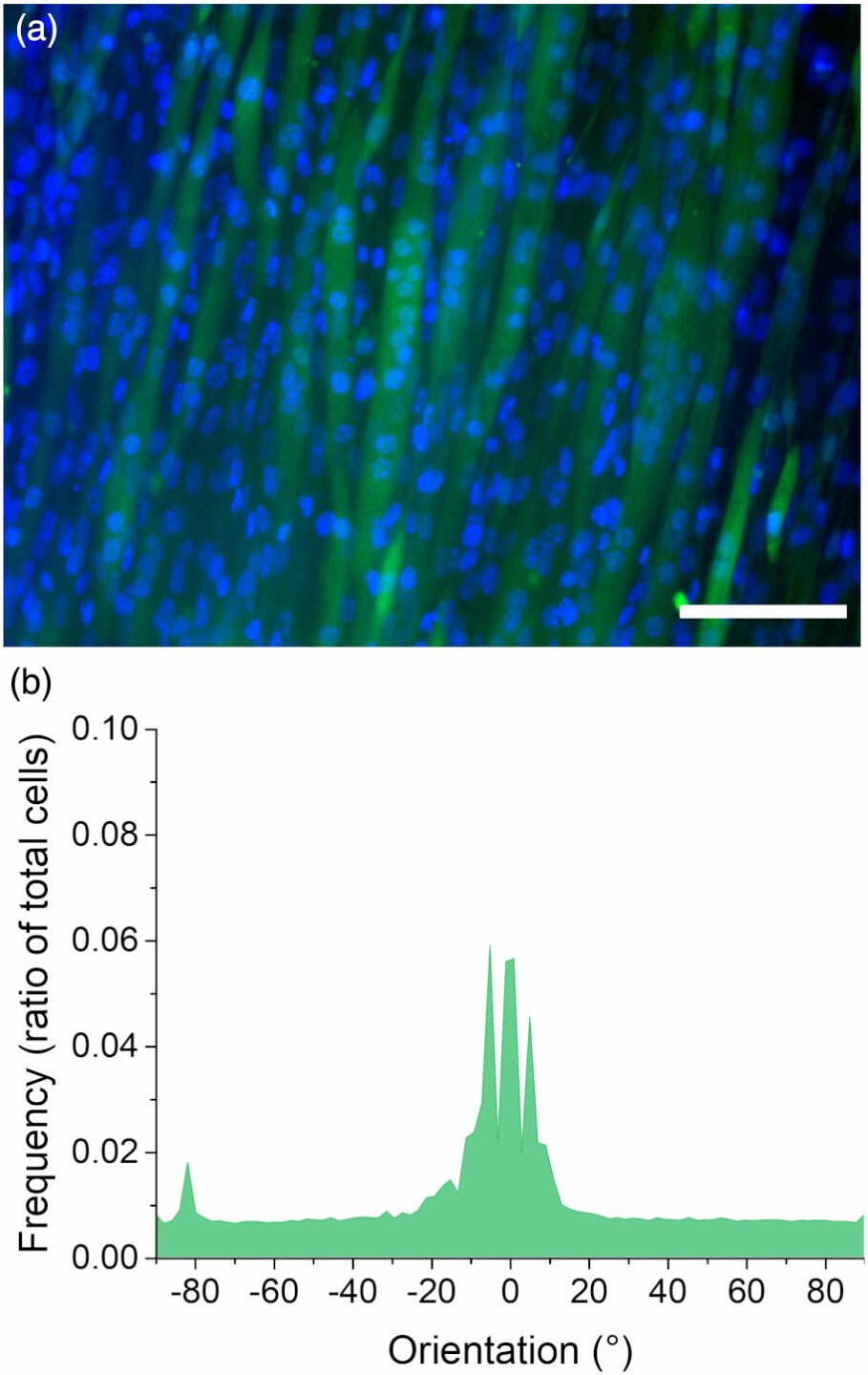
The use of fungal mycelium may also serve as a scalable and affordable scaffolding substrate, without the requirement for decellularization (Figure 13). The company Excel Bio was recently founded in 2019 to explore this possibility. Human control of the species strain in combination with temperature, carbon dioxide, humidity, airflow, or sugar source can enable the growth of predictable structures. Similarly, bacterial nanocellulose (BNC) may exhibit some of the same properties as cellulose-rich decellularized plant scaffolds. A recent investigation of the BNC scaffolds developed by Cass Materials for use in cultivated meat revealed that they were capable of supporting adhesion and differentiation of C2C12 cells, though it was also noted that further optimization was needed to improve cell retention and long-term survival (Rybchyn et al. 2021). Thus, by borrowing techniques pioneered for human organ generation and leveraging the plant, fungal, and bacterial kingdoms for practicality, using prefabricated scaffolds by nature may be the most likely to take hold in the cultivated meat industry. GFI is supporting several research projects focused on using materials from plants and similar sources in scaffolding, including those led by Dr. Masatoshi Suzuki, Dr. Amy Rowat, Dr. Aline Bruna da Silva, and Dr. Vivian Feddern.
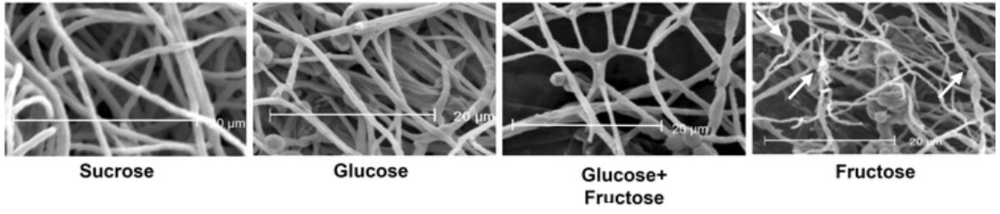
Enhancing differentiation and maturation
In general, deriving cells from stem cells in vitro results in a variety of immature phenotypes such as size, shape, gene expression, and function, which collectively are more akin to a fetal state versus that of an adult. This phenomenon is most apparent under 2D culture conditions where a cell is restricted in 3D space, resulting in alterations in ECM-mediated mechanotransduction, cell polarity, and cell-to-cell interactions (Tibbitt and Anseth 2009). Additionally, exposure to cell culture media containing homogeneously distributed growth factors as opposed to dynamic spatial gradients can influence a variety of cellular behaviors (Ashe and Briscoe 2006). For these reasons, the further maturation of cells in vitro has been an important area of research for stem cell biologists, with consequences for structured cultivated meat products. For instance, immature myotubes may not contain the adult sarcomeric protein content needed for matched nutrition or texture to muscle from an adult animal (Listrat et al. 2016). The methods previously discussed all aim to replicate the 3D in vivo environment in which cells grow, primarily through biomimicry of the ECM. Indeed, transplantation of stem cell-derived cells into an animal is the most well-characterized method to increase functional maturity (Incitti et al. 2019). However, additional methods can be utilized for the maturation of cells for cultivated meat production, discussed below.
In vivo, skeletal muscle fibers and their collagen-rich ECM are highly aligned and thus anisotropic. Therefore, in addition to mimicking porosity and other features of the ECM, growth of skeletal muscle on or within micro or nanopatterned topography can align, polarize, and increase the maturation state and functional output of myotubes (Kim et al. 2012; Orellana et al. 2020; Takahashi et al. 2022). However, mathematical modeling and lab-scale experimental data suggest that the expansion of cells in microchannels can limit oxygen diffusion (Jaques et al. 2021). Similarly, the growth of C2C12 myoblasts on curved substrates improved alignment and myogenic differentiation relative to planar substrates (Connon and Gouveia 2021). The shear forces created during extrusion bioprinting can also be leveraged to enhance alignment. Ahrens et al. (2022) used bioprinting of pre-aligned organ building blocks to enhance both large- and small-scale alignment in engineered cardiac muscle tissue. For electrically active cells such as skeletal myotubes, stimulation can lead to well-described downstream gene transcriptional changes that can influence fiber type composition, maturation, and hypertrophy (i.e. growth, Gundersen 2011). Electrical stimulation of skeletal muscle (Video 4) is mediated via the neuromuscular junction in vivo, however, in the absence of motor neuron input, similar effects can be accomplished via electrical pulse stimulation (Ito et al. 2014). It is also possible to combine multiple strategies for improving myogenic differentiation and maturation. For example, applying electrical stimulation to anisotropic scaffolds further increased myotube width, area, and length beyond what was achieved using anisotropy alone (Zhang et al. 2021).
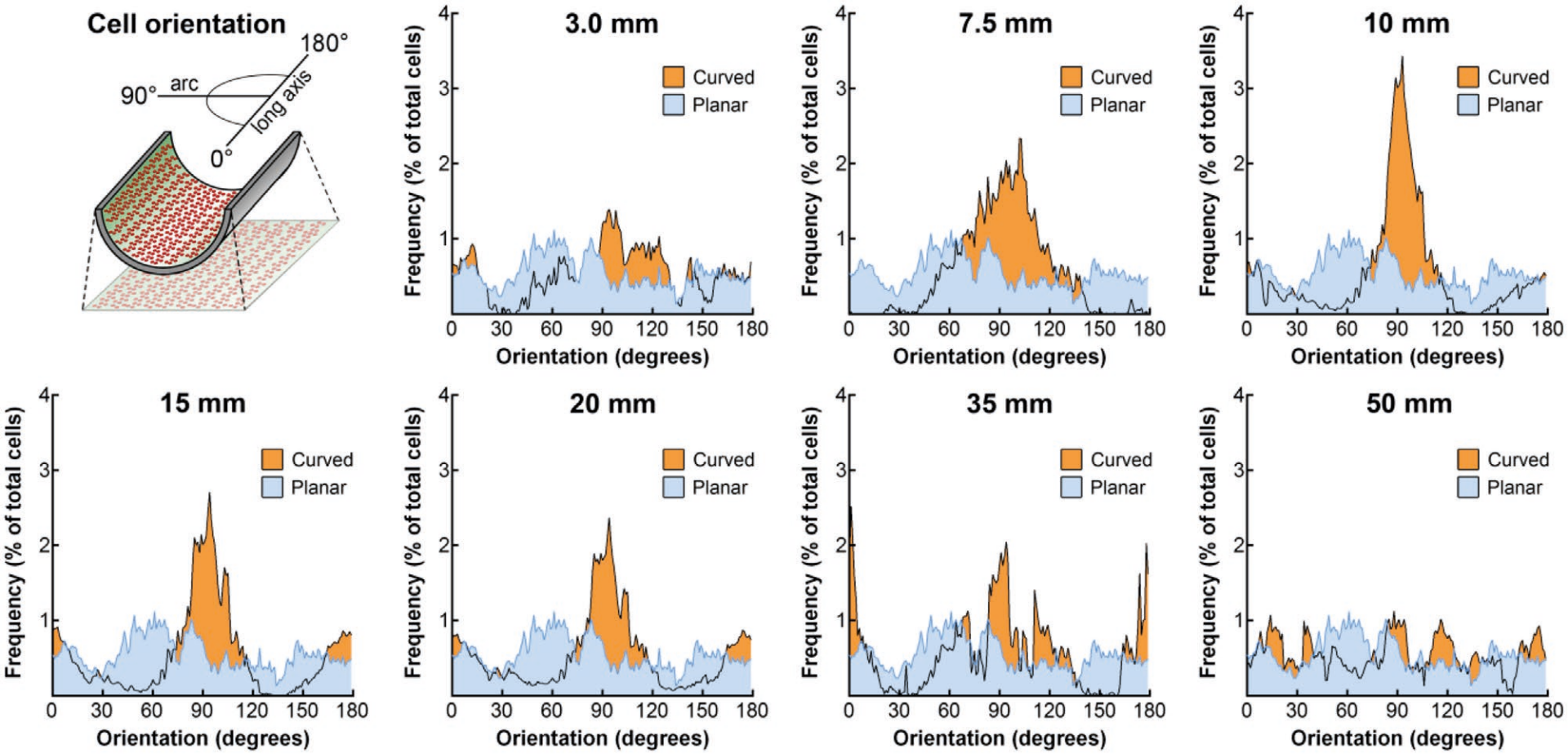
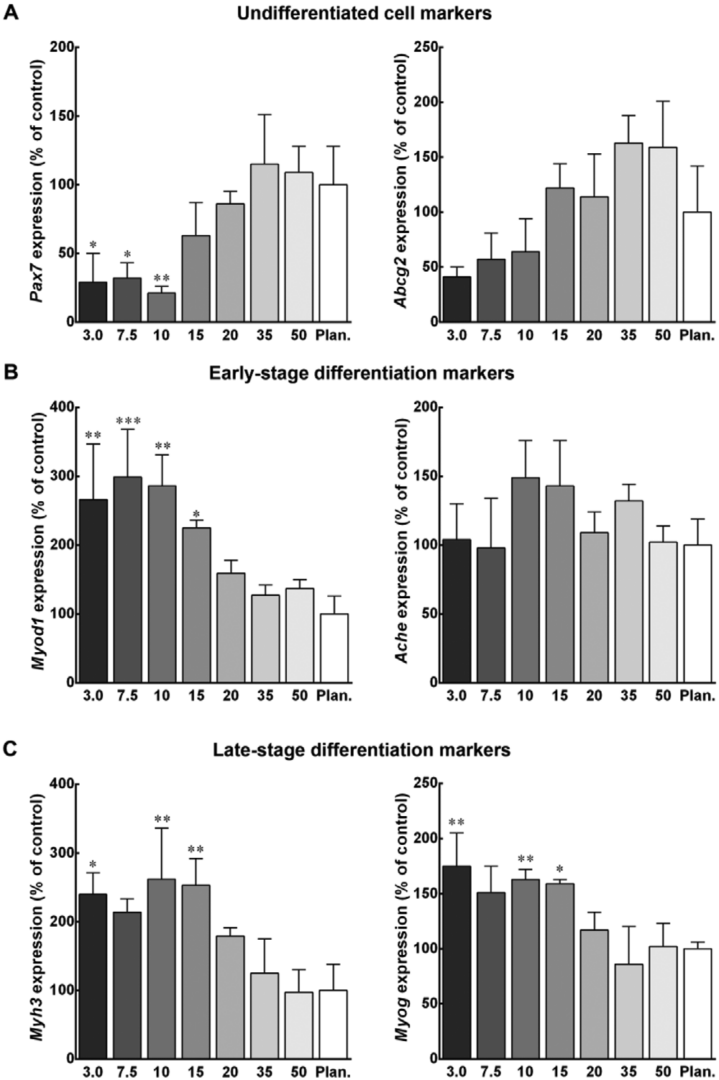
Additionally, myotube growth on conductive polymers such as polyaniline (Jun et al. 2009), conductive coatings such as gold or titanium (Yang et al. 2016), or in the presence of a static magnetic field (Coletti et al. 2007), can assist in myotube maturation in part via myotube alignment and propagation of intracellular calcium signaling through conductive surfaces or gap junctions (Coletti et al. 2007). Likewise, the presence of motor neurons within a 3D bioprinted scaffold containing myotubes resulted in improved myotube formation and maturation, presumably due to functional neuromuscular connections being made (Kim et al. 2020). Lastly, many stem cell-derived populations of myotubes will spontaneously contract upon the development of sarcomeric structures that mediate contractility. In essence, the ability to “exercise” skeletal muscle in vitro is an important determinant in the overall size and maturation state of the cells, as demonstrated through its use in the production of the first cultivated meat burger. In theory, it may be possible to use a continuous or phasic stretch stimulus parallel to the grain of a growing cultivated meat product to simultaneously provide an in vivo-like exercise stimulus and induce lengthwise growth of the tissue, thereby allowing for sequential harvests of meat. However, this hypothesis remains to be tested.
However, many studies investigating muscle contraction in vitro are nascent and at small scales (often intentionally in the biorobotics field) (Ricotti et al. 2017), and have used structured pillars to act like tendons to assist in force generation (Cvetkovic et al. 2014) rather than structured collagen and sparse fibroblasts as may be seen in vivo or used within a scaffold. Thus, the selection of a strategy for ‘exercising’ cultivated meat within a bioreactor at large scale or within a scaffold may be challenging and require new innovations. For instance, direct electric current application to the cell culture medium can lead to ionization of medium components, and the intensity, frequency, and periodicity of stimulation will need to be optimized (Pascoal-Faria et al. 2019). Lastly, there is some concern for the structural integrity of a scaffold to withstand forces generated upon contraction; however, the forces generated thus far from muscle constructs in vitro are orders of magnitude lower than skeletal muscle in vivo (Ricotti et al. 2017) and are unlikely to pose significant problems for applications in cultivated meat.
3d microenvironments
One interesting approach to scaffolding for cultivated meat could be the use of microfluidic spinning (Onoe et al. 2013) or coaxial microextrusion (Li et al. 2018) to produce cells encapsulated in a hydrogel core-shell tube structure, otherwise known as a 3D microenvironment. These techniques work by co-extruding cells within a hydrogel that can be readily crosslinked, such as alginate in the presence of calcium. By creating cell-laden tubes with a 400µm diameter, for instance, cells can readily proliferate within a 3D microenvironment without suffering from mass transport limitations, as oxygen, nutrients, and waste can pass through the porous hydrogel. Additionally, cells are shielded from shear forces within a bioreactor, remarkably high densities of up to 5×108 cells/mL of microspace can be achieved, and differentiation can be achieved via changes in culture media (Video 5, Figure 16, Lin et al. 2018). Maturation can occur inside the core-shell tube itself (Hsiao et al. 2015), enabling an entire process of proliferation, differentiation, and maturation to occur inside a single unit. This effectively bypasses seed-train scale-up or switching of bioreactor formats during the bioprocess.
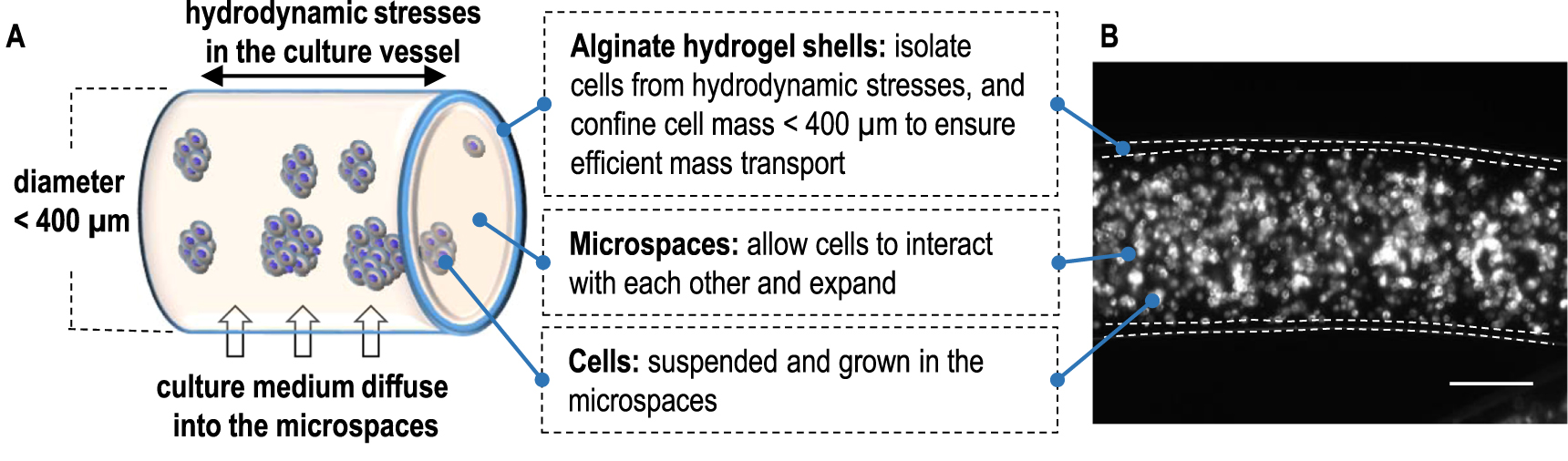
The creation of 3D microenvironments is scalable for both tube length and parallelization of extruders. The tubes are flexible and structurally sound, permitting handling via ejection or suction and can be woven into complex structures using tools and methods similar to the highly automated textile industry (Onoe et al. 2013). Furthermore, alginate can be dissolved in the presence of calcium chelators or enzymes, resulting in a purely cellular structure following handling. 3D tubular microenvironments have been used to grow various cell types, including neurons (Lin et al. 2018), skeletal and cardiac muscle, and adipocytes (Hsiao et al. 2016). Different microenvironment shapes, such as spheres produced using a microfluidic device, have also been produced by cellular therapeutics company TreeFrog Therapeutics (Video 6). A textile-based approach has also been demonstrated using harvested mixtures of fibroblasts and extracellular matrix that could be knitted, crocheted, braided, woven, and wound (Magnan et al. 2020). Thus, these strategies may provide non-obvious solutions for tackling various challenges associated with scale-up and the creation of structured products.
Effects on end product properties
Besides acting as a support for cells during the culture process, scaffolds may also directly impact the properties of the end product (Ng & Kurisawa, 2021). For example, plant-based scaffold materials could contribute naturally-occurring dietary fiber or other components with positive effects on the nutrition profile of the final product. Scaffolds could also serve as delivery mechanisms for nutritionally-important compounds such as long-chain omega-3 fatty acids. The scaffold may also be important for determining the fat content and distribution and the species-specific properties of the end product. Any remaining scaffold material in the product will influence its flavor, texture, and other organoleptic properties. However, the properties of that remaining material may differ from those of the starting scaffold due to interactions between the cells and the scaffold during the culture period. For example, Lee et al. (2022) found that gelatin/agar-coated TVP, while initially stiffer than conventional beef, resulted in a final product with a similar stiffness to conventional beef after being seeded and cultured with myoblasts.
Considerations for perfusion of scaffolds embedded in bioreactors
As described previously in Bioprocess Design, shear stress due to turbulence, bubble ruptures, or differences in slip velocities within a stirred tank bioreactor is a key consideration, as the forces exerted on the cells can influence migration, differentiation, proliferation, and viability. Similarly, perfusion flow through a scaffold to deliver oxygen and nutrients can also generate shear forces on cells. The perfusion flow through a porous structure will generally obey Darcy’s Law of permeability, which describes the relationship between pressure drops related to the length of the flow and the liquid’s velocity (Li et al. 2019). If a scaffold is designed to be embedded within a large volume bioreactor, its size may be limited de facto in order to maintain cell viability due to shear or nutrient mass transfer.
For instance, shear stress due to fluid flows through a scaffold of just a few millimeter thicknesses can influence proliferation, differentiation, and ECM deposition of the cells within the scaffold, as cells tend to respond to shear stresses in 3D differently than in 2D (Zhao, Chella, and Teng. 2006). This is complicated due to the fact that different cell types will respond to shear stresses differently and scaffolds with heterogeneous porosities or mechanical properties will have resulting variable local microenvironments of shear, fluid flow, and thus nutrient mass transfer. Moreover, filling of void space in the scaffold due to cell proliferation and maturation will also have effects on shear, fluid flow, and nutrient mass transfer (Guyot et al. 2015). Computational modeling of fluid flows through different scaffold designs and materials in the presence of different cell types will therefore be needed to inform the rational selection of materials and construction methods for cultivated meat and further the understanding of dynamic cell behavior following scaffold seeding (Maes et al. 2009; Raimondi et al. 2006).
The creation of sensor systems that permit dynamic adjustment of flow rates are also likely to be required. Existing bioreactors for mammalian cell growth may provide some insight into best approaches (Wang et al. 2019), as well as learned principles from solid state fermentation used in production of fungi or plant hairy root culture used to produce metabolites or proteins. Additionally, sterilization procedures for scaffolds such as gamma and UV irradiation or ethylene oxide may denature or damage certain biomaterials, thus limiting certain raw material options (Caliari and Burdick 2016; Dai et al. 2016). Forward-thinking strategies to address all of these challenges should be pursued.
Summary of scaffolds for cultivated meat
Putting this information together, a scaffolding structure for cultivated meat will likely require leveraging multiple aspects of state-of-the-art tissue engineering. A scaffold will likely be composed of cheap, edible or biodegradable biomaterials with high biocompatibility and porosity suitable for cell migration (approximately 1 – 500µm), continuous perfusion, and recycling of cell culture medium to remove waste and refresh nutrient supply. This may leverage native tissue architecture (e.g. decellularization) or be constructed by design (e.g. 3D printing, spinning techniques, core-shell tubes). The scaffold may consist of different composite biomaterials, pre-patterned to recreate the cytoarchitecture of meat, with differing stiffnesses and/or embedded growth factors to assist in stem cell attachment, migration, and maturation into their differentiated counterparts.This may be less challenging than it initially seems, as cultivated meat does not require the same microscale precision and organization required for functional tissues — it merely needs to represent tissue structure sufficiently to recapitulate the appropriate texture and mouthfeel of its conventional counterpart. Alternatively, cells grown in tubular (Li et al. 2018) or thin-sheet (Allen et al. 2017; Takahashi, Shimuzu, and Okano. 2018) structures may be constructed into meat products using additive manufacturing principles.
The level of cellular maturation required will likely be dictated by the final product’s nutritional and textural requirements, and a spectrum of options may be considered in practice. As discussed in End Products, many years will likely separate the release of unstructured versus structured products as described here due to the difficult nature of the process. Significant challenges will need to be met with innovations to address concerns of scaling, cost, and reproducibility, as current practices in tissue engineering are prohibitively expensive and technically challenging. Despite this, current technologies provide a foundational roadmap for the successful creation of future structured cultivated meat products. Scaling up cultivated meat scaffolding will require partnerships between ingredient suppliers and scaffold manufacturers. Scientists in tissue engineering and cultivated meat should be encouraged to share knowledge and collaborate around shared problems, resulting in positive mutual benefits for each respective field.
View references featured in scaffold manufacturing technologies
Scaffolds for seafood
Introduction
The fundamental challenge in developing scaffolds for cultivated seafood is the same as for cultivated terrestrial meat. However, some important biological differences may render different approaches appropriate depending on the species in question. In addition, mammalian tissue engineering has been a key research priority for years due to its biomedical applications. Because the same is not true for fish or aquatic invertebrates, research into appropriate scaffolds for these species is at an earlier stage. Even so, companies and academic researchers are making progress in developing scaffolds for cultivated seafood.
Key differences in biology
Designing an appropriate “spec sheet” for the desired properties of a cultivated seafood scaffold will require understanding several critical biological differences between terrestrial vertebrates, fish, and aquatic invertebrates. A scaffold that works perfectly for beef may not work well for salmon or shrimp because the cells and tissues of these species have different properties and requirements. These considerations can be broadly divided into requirements related to the basic requirements of the cells in question and those related to the properties of the end product. A scaffold designed based on only the first set of considerations might facilitate excellent cell adhesion, proliferation, and differentiation but might produce a final product that is inappropriately tough or otherwise undesirable as food.
Basic requirements
Because open-access research into scaffolding for seafood is still in its infancy, it is unclear to what extent scaffolds developed for use with cells from terrestrial animals will be capable of supporting the growth of fish, crustacean, or mollusk cells. It is well known that matrix stiffness influences differentiation, which suggests that scaffolds for seafood might have different stiffness requirements than those for terrestrial vertebrate muscle. Researchers attempting to design scaffolds for use with fish or aquatic invertebrates should therefore consider varying the scaffold stiffness to find appropriate conditions. As in terrestrial animals, the properties of the ECM are essential for guiding the differentiation process in fish and aquatic invertebrates. For example, the ECM guides myogenic and neuronal differentiation in mussels, with collagen coatings tending to promote proliferation while coatings of fibronectin, poly-L-lysine, or carbon tended to promote myogenic differentiation (Dyachuk 2013; Odintsova et al. 2010).
Requirements related to end product properties
Designing scaffolds that appropriately support the adhesion, proliferation, and differentiation of aquatic cells is only half the battle—scaffolds must also be compatible with the desired organoleptic properties of the final product. Fish muscles differ from those of terrestrial vertebrates and aquatic invertebrates in that they are organized as thin sheets—called myomeres—separated by layers of fat and connective tissue known as myosepta. The distribution of fat, fast muscle fibers, and slow muscle fibers also differs between broad groups and species within each group (Listrat et al. 2016). If the goal for a given product is to replicate the properties of conventional seafood, the design of the scaffold will need to take these differences into account.
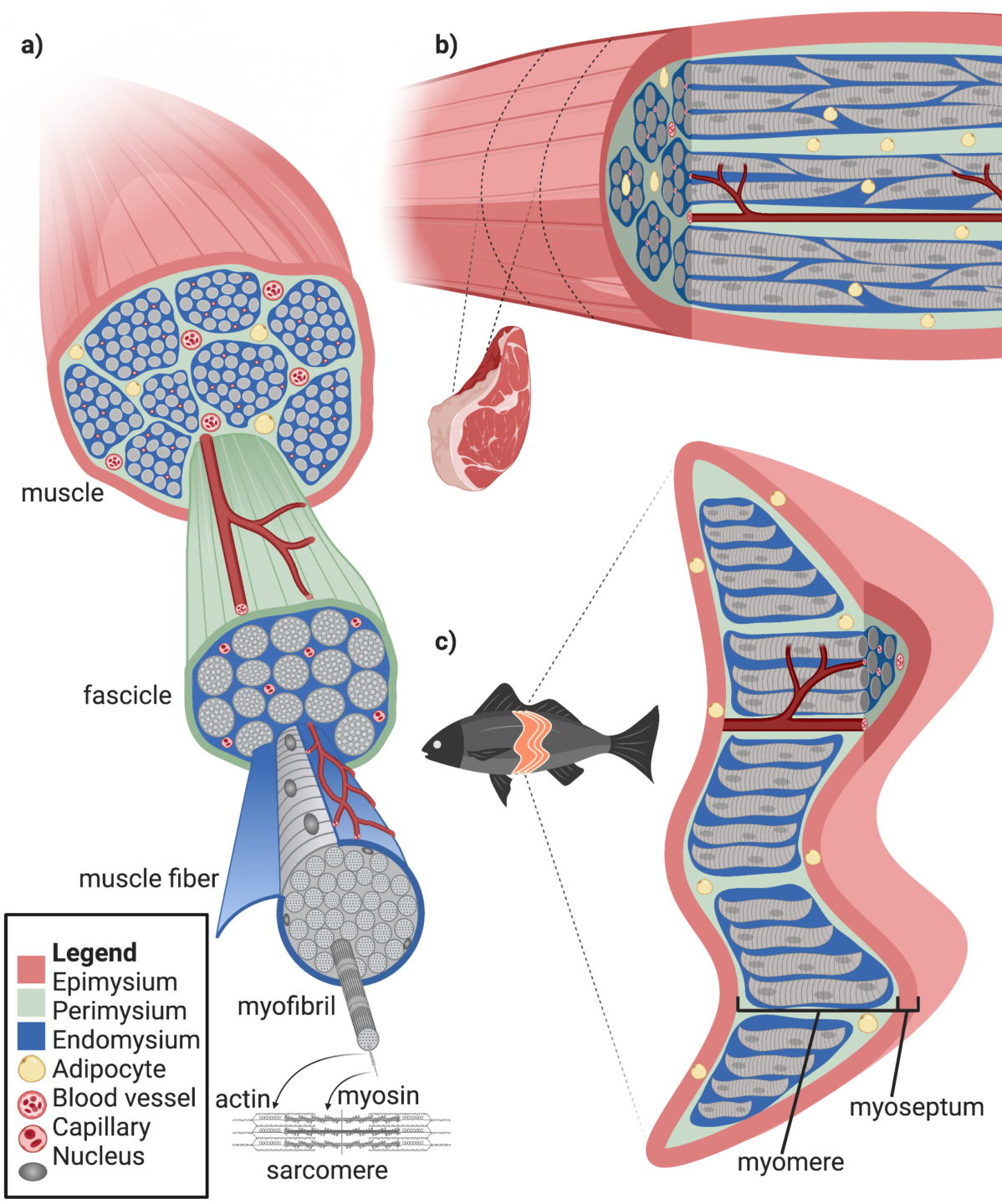
The properties of ECM proteins from terrestrial and aquatic sources differ in ways that are likely to be important for end product properties. One key difference is the lower melting temperature of fish collagen, which contributes to the flakiness of cooked fish. For both terrestrial meat and seafood, any scaffolding material remaining in the final product must not impart negative attributes such as excessive toughness. However, this requirement may become more salient for many types of seafood, which are naturally more tender than most terrestrial meat. That is, a scaffold that remains in the final product and has an acceptable—or even positive—effect on the texture of terrestrial meat could result in an unpleasantly tough seafood product.
Various compounds in the final product will contribute to flavor, color, and nutrition. Some of these are not derived from animal cell metabolism but must be supplied via the animal’s diet in the case of conventional meat or via the cell culture environment in the case of cultivated meat. Such compounds include omega-3 fatty acids, carotenoids, and vitamins such as B12. These compounds could be incorporated into the product through various strategies, including via the scaffold. Because many of these diet-derived compounds are found at dramatically different levels in meat from different species, scaffolds intended for different species might be supplemented with different nutrients. For example, a scaffold designed for salmon might have higher levels of astaxanthin and long-chain omega-3 fats—which can also contribute to the positive aspects of salmon flavor— than one designed for terrestrial meat or for freshwater seafood.
Scaffolding strategies in use by cultivated seafood companies
Several startups pursuing cultivated seafood have announced the general type of scaffold—or other methods for producing structured products—they are using. Wildtype, Wanda Fish, and Finless Foods are reportedly using plant-based scaffolds for their initial products. Finless Foods is also commercializing the same material—the main ingredient in which is winter melon—as a plant-based seafood product. CytoNest—a B2B scaffolding company and a GFI grant recipient—has demonstrated successful growth of fish stem cells on their edible polysaccharide- and protein-based fiber scaffolds.
BlueNalu is taking a different approach by foregoing pre-fabricated scaffolds in favor of extrusion bioprinting. Umami Bioworks, in partnership with Steakholder Foods, demonstrated a 3D bioprinted cultivated grouper prototype. Because this prototype did not undergo a maturation step after printing, its texture presumably would have depended on the materials used in the bioink and on the details of the printing procedure. However, this is not inherently true of 3D printed cultivated products, as the inclusion of a post-printing differentiation or maturation step would allow for the self-organizing properties of the printed cells to contribute to the product’s texture. Forsea Foods also avoids scaffolds, instead using an organoid-based approach to produce muscle, fat, and connective tissue from freshwater eel cells.
For the cell proliferation stage, Wildtype reports that they have used strategies ranging from microcarriers to cell clusters to single-cell suspension (Waltz 2021). Bluu Seafood and Atlantic Fish Co are both using single cell suspension-based approaches for cell proliferation.
Academic research
Academic researchers are also tackling the challenge of scaffold development for seafood. For example, the successful growth of muscle cells from goldfish on microcarriers was recently reported (Dong et al. 2023a). In another study, large yellow croaker satellite cells grown on gelatin microcarriers, 3D gelatin methacryloyl hydrogels, and tissue culture-treated glass exhibited differences in proliferation rate and gene expression, especially genes related to the integrin signaling pathway (Yin et al. 2024). Dr. Shigeki Sugii’s group reported the successful growth and adipogenic differentiation of Pangasius cells on Cytodex-1 microcarriers, but also reported difficulties getting the same cells to grow in spheroids or in suspension.
Decellularized banana leaves were investigated as possible scaffolds for zebrafish cells (Banavar et al. 2024). The use of vacuum seeding shortened the time needed for cell seeding and promoted a more even distribution of cells throughout the scaffold volume. However, the authors also hypothesized that the limited penetration of the culture medium into the center of the scaffold was responsible for the observed issues with long-term (between 24 and 48 hours post-seeding) survival of these deeper-seeded cells. Coating these scaffolds with soy protein or gelatin did not impact the efficiency of cell seeding, but did impact cellular alignment, proliferation, and differentiation. Both coatings increased alignment, and the soy coating also appeared to delay differentiation and increase proliferation. The authors also investigated the behavior of empty and seeded scaffolds during cooking and found that their thermal stability was slightly higher than that of conventional seafood. While further optimization is needed, this represents a promising starting point for the goal of producing scaffolds that are both biocompatible and appropriate for the intended food application.
Preliminary work on hydrogel scaffolds for cultivated fish has also been reported, including work by Dr. Mark Richards and his team on self-assembling dipeptides and microbial polysaccharides. The utility of fish collagen for cultivated seafood scaffolding has also been investigated, with self-assembled hydrogels from Australasian snapper collagen as well as fiber scaffolds produced from the denatured collagen of either Australasian snapper or Chinook salmon showing promise (Stent 2024).
A recent paper described the use of a bioink consisting of fish gelatin and alginate to print small-scale prototypes of cultivated large yellow croaker muscle that mimicked the structure and fiber orientation found in naturally-occurring myomeres and myosepta (Xu et al. 2023). The bioprinted constructs were able to support cell proliferation and differentiation, and following the differentiation phase, the prototypes were fairly similar to conventional fish in terms of their microstructure and textural properties. A subsequent paper by the same group showed that adding starch nanoparticles to the bioink improved printability, cell adhesion, viability, and proliferation rates (Niu et al. 2024). The constructs printed from ink containing the nanoparticles showed better cell alignment compared to controls following 12 days of proliferation. After a further 5 days in differentiation medium, they also showed higher rates of cell fusion and formed thicker and more organized cell fibers.
An ongoing project at the University of Lisbon aims to combine bioprinting—to create the striated geometry of fish muscles and myosepta—with electrospinning to create the fine-scale alignment of the individual muscle fibers. The Algae2Fish project recently published its first report, in which the researchers characterized their κ-carrageenan-based bioink and identified conditions that improved printability (Marques et al. 2022). This project also investigated the addition of plant-derived materials to enhance the electroconductive properties and color of the bioinks (Marques 2021). Subsequent work from the same group focused on 3D bioprinting as a strategy for producing adipose tissue from European seabass cells (Jabouille 2023). The inclusion of microalgae and omega-3 fatty acids in the bioinks was also explored. A mostly scaffold-free method for cultivated fish production was also reported, in which cell sheets grown on a collagen-coated surface were detached from the substrate and layered to form the product prototype (Tsuruwaka and Shimada 2022).
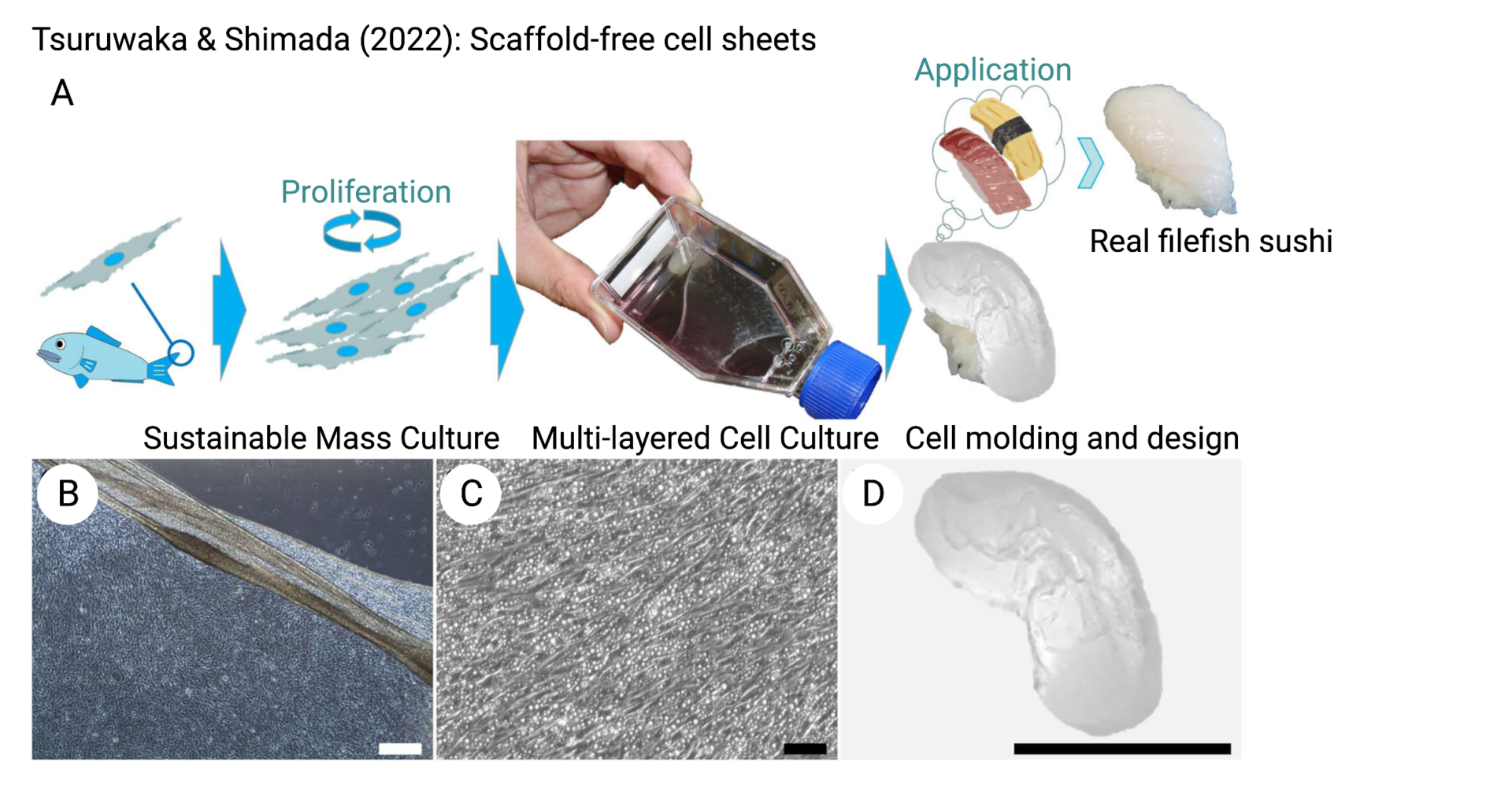
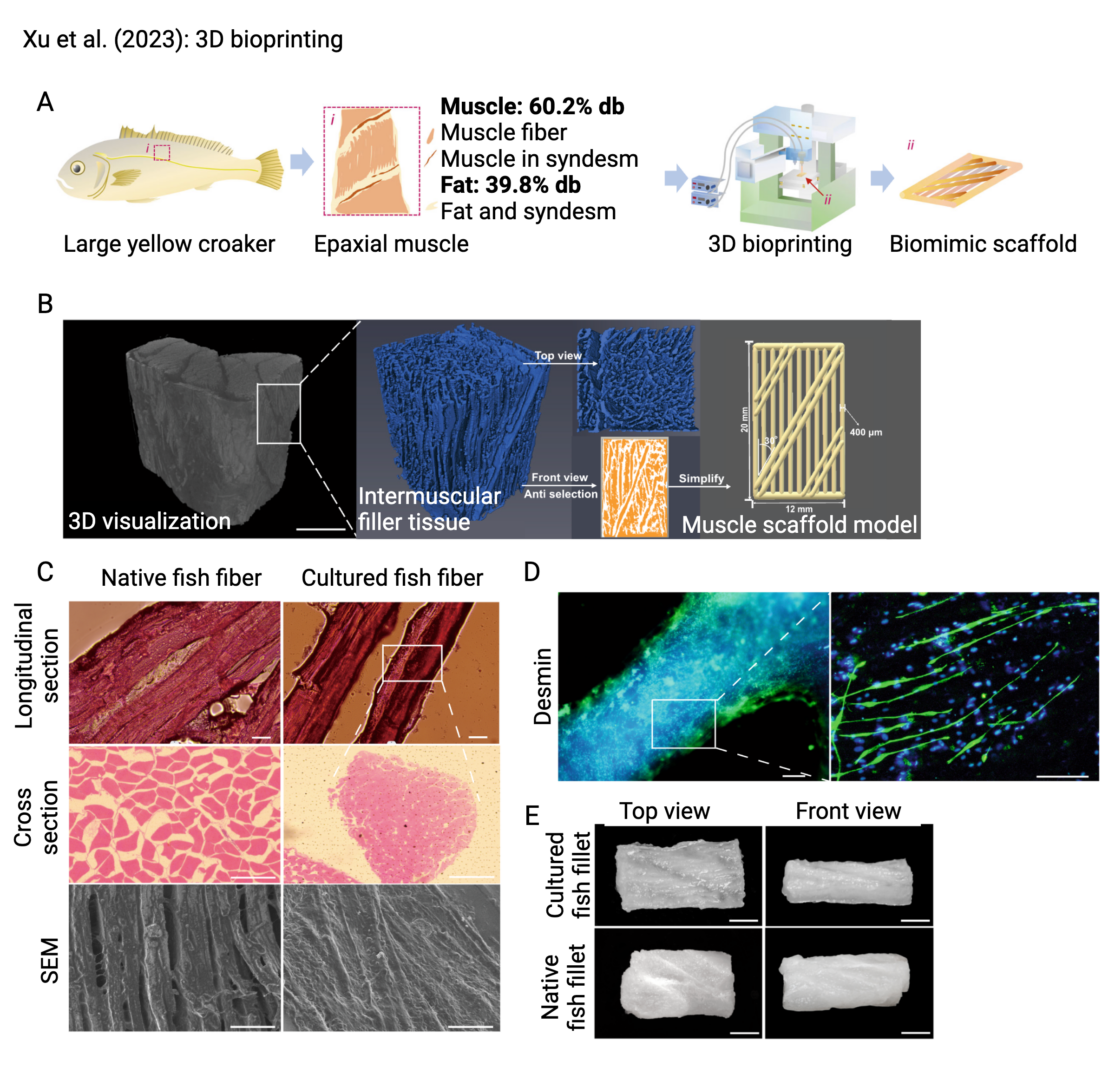
Research into scaffolds for aquatic invertebrates has thus far been quite limited. A likely contributing factor is the lack of appropriate cell lines from food-relevant invertebrate species. For example, Cytonest’s GFI-funded project (mentioned above) initially focused on scaffolds for shrimp, but the team later pivoted to work on fish due to challenges accessing an appropriate cell line. Because of the close evolutionary relationship between insects and crustaceans, the scaffolding requirements between the two taxa may be somewhat similar. Unsurprisingly, research into insect cell scaffolding is similarly at an early stage, though some preliminary studies have been published. Drosophila muscle progenitors were successfully grown and differentiated on scaffolds produced by directional freezing of fungal chitosan (Rubio et al. 2019). According to Dr. Danai Georgiadou, tiger prawn satellite cells tend to prefer growing in suspension, suggesting that the use of microcarriers or spheroids during the proliferation step may be unnecessary for some crustacean cells.
There remains a substantial need for open-access research to adapt existing scaffolding technologies—or alternatives to scaffolding—for cultivated seafood’s biological and organoleptic requirements. Collaboration between tissue engineers, fish cell culture experts, and food scientists will be essential in moving this research forward.