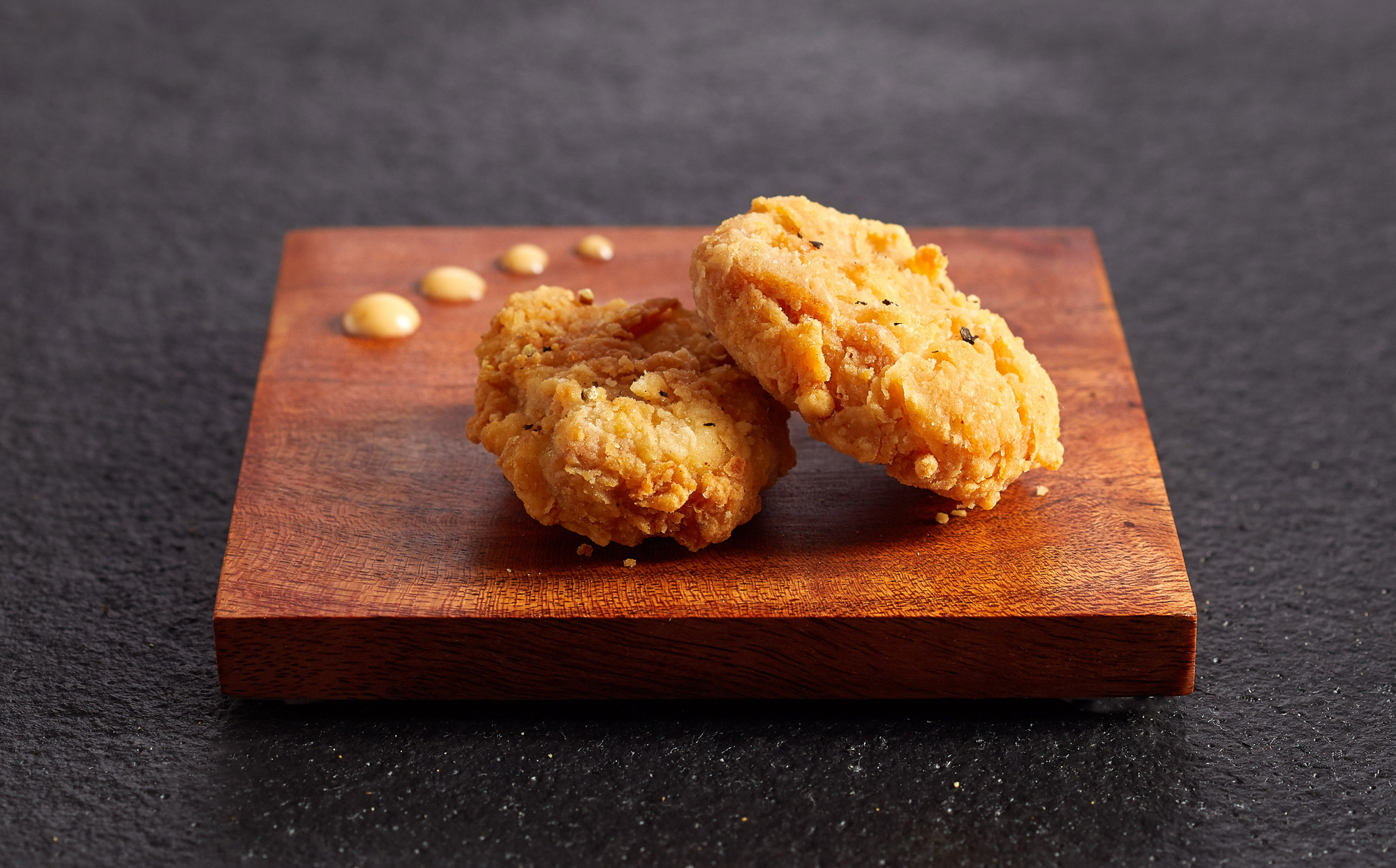
Deep dive: Cultivated meat bioprocess design
Learn about the bioprocess design parameters critical to the development of cultivated meat.
Bioprocess design
Introduction
If the goal of cultivated meat production is to significantly reduce the levels of meat consumption from industrial animal agriculture and in turn reduce the associated negative impacts, then large quantities of meat will need to be produced affordably and efficiently. The first cultivated meat product was sold in Singapore in December, 2020, with several other cultivated meat prototypes taste-tested, including duck, chicken, salmon, yellowtail, shrimp, pork sausage, foie gras, fish maw, fat, beef meatballs, beef hamburgers, and beef steak strips, amongst others. However, moving from bench-scale methods to pilot scale and commercial scale production requires an influx of talented scientists from a wide array of disciplines in order to solve significant technical hurdles in cell biology and engineering optimizations. These hurdles, potential strategies for solutions, and the roadmap to scaling cultivated meat are discussed below.
Bioreactors
Although cultivated meat products for taste testing have been produced using standard cell culture dishes and stacked flasks, growing cultivated meat at scale will require the use of bioreactors in volumes up to or beyond several thousands of liters. This fundamental switch in how cells are grown brings in additional considerations such as gas exchange, heat transfer, shear stress, mixing, and foaming, which are not of common concern to the standard cell culture technician. While many of these techniques can be borrowed from industries such as cell therapies, recombinant protein production, or production of other biologics, considerable optimizations are required in order to move cultivated meat beyond taste-testing events and toward market readiness. This will require an interdisciplinary effort amongst cell biologists and chemical, mechanical, and bioprocessing engineers.
A variety of methods can be selected for scaling cultivated meat production past bench into pilot and commercial scales. Broadly speaking, these methods can be broken up categorically into batch, fed-batch, continuous, and perfusion (Meyer, Minas, and Schmidhalter 2017). In batch culture, a vessel is filled with a fixed volume of media and cells are grown to their maximum density before being harvested or transferred to a larger vessel. In fed-batch culture, cells grown in a vessel are fed fresh medium from an in-line, independent feed vessel at variable rates in order to maximize properties such as exponential cell growth or cell densities. In continuous culture, cells are grown in a vessel and fresh medium is added via an in-line feed vessel at an optimized flow rate, while product, cells, or medium are simultaneously collected in an independent collection vessel at the same or alternative rate. Lastly, perfusion culture is a subset of continuous culture wherein the cells are retained via a substrate or collection method, permitting medium recycling integration and high cell densities in a smaller space. Each method has potential benefits and caveats, and it is possible that multiple methods may be used throughout a cultivated meat bioprocess.
There are many different bioreactor designs to choose from that can be separated based on how the medium is mixed and whether the cells are grown in suspension or adhered to a solid surface. For animal cell culture, the most commonly used bioreactor is a continuous stirred tank reactor, as it offers greater long-term sterility and reduced bubbling versus air-lift reactors at scale (Meyer, Minas, and Schmidhalter 2017). In general, continuous stirred tank reactors permit growth of cells in suspension via mechanical stirring while maintaining high mass transfer of oxygen. Similar suspension growth results can be obtained with rocking platform bioreactors and vertical wheel bioreactors, albeit at smaller maximum scales. Suspension growth can also occur with attachment-dependent cells through the use of microcarriers (discussed later).
Current cell therapy and biopharmaceutical industry trends show preference for stirred tank and rocking platform bioreactors in disposable, single-use systems up to 6000L. Single-use bioreactors have the advantage of not requiring heated sterilization (discussed later), saving on turnaround time, cross-contamination, water, energy, and sensor (discussed below) costs. Therefore, single-use bioreactors may be a favorable option when considering scale-out methods (discussed later) of production, however the comparably lower value of a batch of cultivated meat product versus a biologic drug or human cell therapy means that single-use technologies would likely not make economic sense for cultivated meat unless current models can be made more affordable. Notably, however, single-use bioreactor bags consist of various material layers that must meet strict regulatory standards at high cost in order to ensure that a high-value batch of product is not lost. Thus, at lower batch value and food-grade regulatory requirements, there may be room for engineering economically-feasible, food-safe disposable bags tailored for cultivated meat production. The favorable sustainability metrics of single-use bioreactors that show savings on water and energy usage from sterilization compared to stainless steel bioreactors will have to be weighed against the waste that is produced from single use.
In biopharma, stirred tank bioreactor vessels have been used for animal cell production in volumes up to 20,000L (Li et al. 2019). However, these volumes may not be sufficient for cultivated meat, which must achieve production volume more akin to the food and feed industries rather than biomedicine. Air-lift reactors also permit suspension growth and become advantageous at very large scales (e.g., >20,000L), as their mixing does not involve moving parts, leading to less inhomogeneity, less shear stress (discussed later), fewer nutrient or oxygen gradients, and lower power requirements to perform the mixing (Merchuk 1990). The largest bioreactor ever built was an airlift reactor that held 1,500,000L for microbial cell growth. This airlift reactor design was recently scaled down to 300,000L and evaluated using computational fluid dynamic models for the optimization of reactor geometry and internals for animal cell growth. This resulted in a theoretical cell density of 2×108 cells/mL and a single reactor that could feed 75,000 people annually (Li et al. 2019). Thus, air-lift reactors may offer a more tractable solution for achieving very large scales. However, the dearth of knowledge of animal cell cultivation using air-lift reactors leaves many gaps to be filled before such a scale is realized. Considerably more work in modeling and empirical data collection for animal skeletal muscle, fat, and other cell types will need to be performed using air-lift reactors. As such, much of the focus of the remaining discussion will rely on stirred tank bioreactors where data is more readily translatable.
Optimizations
There are many important variables that can affect a bioprocess when scaling. These include efficiency of mass transfer, avoidance of inhomogeneities, heat dissipation, impeller shape and speed, dissolved oxygen (discussed later), and reactor geometry, amongst others. Each can be affected differently depending on the cell type, scale, and intended downstream use. Adjustments for these variables are likely to be adapted from existing platforms from the cell therapy and biologics fields or assisted by novel engineering and custom-tailored to a specific bioprocess, species, and/or cell type. Thus, the most generalizable optimizations or considerations are discussed below.
Shear stress
One important consideration when scaling animal cells is how to deal with shear stress. Shear stress is the mechanical force induced by the friction of liquid on a cell’s surface (Nerem 1991). Animal cells are generally more susceptible to shear stress than their microbial counterparts due to the lack of a cell wall. In a bioreactor, shear stress can be caused by the liquid’s turbulence created by the impeller (or general motion) in order to keep the cells in suspension. Larger volumes generally imply stronger shear forces, although the force is inhomogeneous throughout the bioreactor, and multiple eddies of turbulence may be generated depending on the impeller type and number (Papoutsakis 1991). Shear stress can impact cell viability (Hu, Berdugo, and Chalmers 2011) and differentiation (Stolberg and McCloskey 2009), but can also be mitigated by installment of flow breakers, cell adaptations, or addition of poloxamers to the culture medium (D. Chang et al. 2017).
The presence of bubbles and their rupturing can also lead to shear stress due to differences in slip velocities. In cells grown in suspension, smaller bubbles (< 1 mm diameter) can lead to higher shear stress and rates of cytotoxicity (Nienow 2006), but this may not hold true for cells grown on microcarriers (discussed later). This opens up the possibility that an air-lift reactor that relies on the controlled bubbling of gasses could be used for animal cell growth if microcarriers are used (Li et al. 2019). The growth of cells in aggregates or on microcarriers can independently influence the subjected shear forces on cells (Figure 1). Tolerable levels of shear stress should thus be calculated for each cell line and cell type (King and Miller 2007).
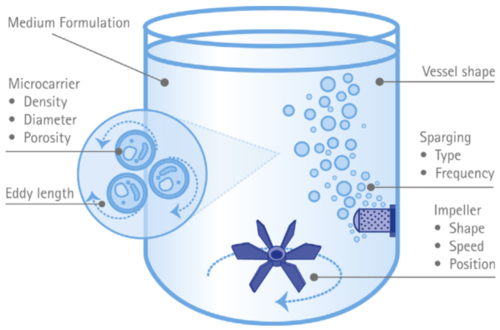
Figure 1. Some of the many factors that can influence cell exposure to hydrodynamic forces in a stirred-tank bioreactor. From Schnitzler et al. 2015.
One potential way to minimize shear stress in stirred tank bioreactors is to produce methods that decrease or eliminate turbulence, resulting in laminar flow. This can be achieved by the creation of novel impeller designs that rely on upward liquid flow that is expelled to the sides of the vessel, effectively allowing the fluid itself to do the mixing and eliminating shear forces over time. These strategies and others (discussed later) may be necessary to scale the production of cultivated meat.
Anchorage dependence and cell adaptation
The vast majority of cell types, including those used in cultivated meat, are anchorage-dependent, meaning that they require some substrate to grow on in order to prevent a form of programmed cell death, termed anoikis. While pluripotent stem cells can be grown as anchorage-independent aggregates in suspension, they must also be routinely dissociated to single cells in order to prevent spontaneous differentiation (Shafa et al. 2012; Zweigerdt et al. 2011), and are typically treated with an inhibitor of Rho Kinase (i.e. ROCK inhibitor) to prevent death as single cells. Pluripotent-derived cell types used in cultivated meat production are largely anchorage-dependent, although recent advances in organoid culture have led to the development of suspension-based differentiation protocols to create an array of iPSC-derived cell types, including skeletal myotubes (Jiwlawat et al. 2017) and sustainable pools of non-committed myogenic progenitors (Mavrommatis et al. 2020). Recent suspension protocols deriving gastruloids demonstrated the creation of somites, progenitor structures that lead to the fat, connective tissue, skeletal muscle, tendons, cartilage, and bone that may serve as a building block for cultivated meat (Rosado-Oliviery and Brivanlou. 2020). These protocols may also better recapitulate the physiology of in vivo cellular counterparts, however, they have limited testing past bench scale (Kim and Kino-Oka 2018). Lastly, aggregate-based culture may be more susceptible to shear stress (Chapman et al. 2014), resulting in lower cell viability and lower cell densities relative to single-cell suspension culture, although these limitations can likely be overcome (Lipsitz et al. 2018).
Adult stem cell types such as myosatellite cells and mesenchymal stem cells are also anchorage-dependent, and are frequently grown in suspension on microcarriers in order to bypass this limitation, although spheroid cultures are a promising alternative (Alimperti et al. 2014; Jossen et al. 2018). However, there are no inherent biological limitations to suspension adaptation. For instance, the adherent epithelial Chinese Hamster Ovary cell type used extensively in the production of biologics has been adapted to suspension growth and optimized for specific traits such as maximized protein production over time in vitro. In contrast, mesenchymal stem cells used as cell therapies are often autologous in nature, requiring minimal scaling and no cell line optimization prior to re-entry into the body (Galipeau and Sensébé 2018). The development of off-the-shelf allogeneic products that necessitate large scale bioprocessing (e.g. 1 million doses) and optimization using established cell lines is still in its infancy. Cultivated meat will command adaptation strategies similar to those in the biologics field using Chinese hamster ovary cells versus minimally-manipulated autologous mesenchymal cell therapies.
A variety of options exist for cell adaptation, including natural or assisted selection, directed evolution strategies (Tizei et al. 2016), or genetic engineering (Lee et al. 2016). Notably, species differences may also determine the likelihood of suspension growth, exemplified by efficient adaptation to suspension growth of insect myoblasts (Rubio et al. 2019) and shrimp cell lines, according to scientists at Shiok Meats. Some immortalized cell lines, depending on the method of immortalization, can acquire anchorage-independence, permitting growth in suspension (Kovalevich and Langford 2013; Qu et al. 2015). Lastly, in adipocytes, the accumulation of lipid droplets upon maturation and resultant increased buoyancy may pose additional problems for cultivated fat production in conventional stirred tank bioreactors (Zhang et al. 2000).
Paracrine factors and metabolites
As cells grow, they release autocrine and paracrine factors that can serve as pro-growth or inhibitory signals to neighboring cells. Under high cell densities intended for cultivated meat production (i.e. > 1×107 cells/mL), these signals can greatly influence downstream proliferation, differentiation, and viability. Computational in silico modeling can assist in the selection of bioprocessing methods by modeling accumulation of paracrine signals and predicting methods to yield favorable outcomes (Csaszar et al. 2012). Identified undesirable signals may also be removed from the culture via filtration whereas desirable signals can be recycled. Alternatively, growth of cells in high concentrations of metabolites such as lactic acid, ammonia (Schumpp and Schlaeger 1992), and low concentrations of growth factors can select for cells tolerant to high densities and lower growth factor requirements (e.g. serum-free conditions) (Sinacore, Drapeau, and Adamson 2000). High-density growth may also require nutrient alterations to the cell culture medium, as well as addition of oxygen carriers (Ozturk 1996) and anti-foaming agents (Velugula-Yellela et al. 2018). It will therefore be important to generate optimized cell lines across a variety of species that are uniquely adapted to the biophysical environment within bioreactors and tolerant to relevant paracrine factors or secreted metabolites during the proliferation and differentiation stages, respectively. Additional research is needed to characterize the secretome of fat and skeletal muscle cells from different species used in cultivated meat.
One example of optimized cell lines for toxic metabolites is a recent patent released by UPSIDE Foods that describes cell lines containing a genetically encoded glutamine synthetase, which enables intracellular conversion of glutamate to glutamine while consuming ammonia in the process. Thus, ammonia can be reduced by ~20% while generating energy substrates for the cell in the form of glutamine (Genovese 2019). Some avian species have been shown to metabolize ammonia differently than mammals in their skeletal muscle due to differences in glutamine synthetase expression, yielding larger myotubes presumably from increased glutamine utilization (Stern and Mozdziak 2019). Thus, inherent species differences also come into play when considering optimizations around the biochemical and biophysical environments within bioreactors.
Process scale down and computational modeling
Because optimizing a scale-up can require large capital expenses, use of high throughput miniature bioreactors can assist in modeling batch or fed-batch processes (Rameez et al. 2014). These “scale-down” approaches allow for users to save on costs while optimizing the parameters that can guide growth at larger scales. However, miniature perfusion bioreactors requiring microfluidics to match flow rates in small volumes has lagged behind and may be more difficult to accurately model at small scales (Fisher et al. 2019). Accordingly, products for high throughput modeling of perfusion methods still deal with volumes of up to 250 mL.
Parameter optimization and other bioreactor design considerations can also be assisted by computational modeling. Computational models reproduce some aspects of the behaviors of a complex system and make predictions under new conditions. This can greatly improve the speed of iteration and breadth of parameter search space compared to performing empirical experiments with burdensome costs and time, as in cultivated meat development (Kahan et al. 2020). Both agent-based models and computational fluid dynamics models can be utilized or even merged to assist in cultivated meat bioprocess and bioreactor design and optimization, as well as other considerations such as media optimization, scaffold design, and end-product characteristics. The Cultivated Meat Modeling Consortium, an interest group involving life science companies, academic researchers, cultivated meat companies, nonprofits, and other stakeholders, was formed in 2019 to accelerate the pace of research and development in cultivated meat by leveraging computer modeling.
Seed trains, continuous processing, and cell density
In order to produce large numbers of cells in large volumes, a progressive scaling process is started from a single vial of cells taken from the working cell bank. This process is called a seed train. As cells grow, they proceed through a lag phase, where the cells adapt to their growing conditions, followed by a log phase where cells proliferate exponentially, and ending in a stationary phase. Thus, the starting density of cells compared to the volume in which they are thawed can influence their access to available nutrients and time to reach log phase (Frahm 2014). This can vary by cell type and species (Cheng et al. 2014). The seed train strategy relies on cells growing exponentially over time, tracked by population doublings, while transferring cells into progressively larger vessels. By the exponential nature of this process, the greatest efficiencies are achieved at high numbers of population doublings. However, a higher number of population doublings can negatively affect downstream proliferation rates and differentiation capacities, as has been observed in a variety of stem cell types (Park et al. 2008; Bonab et al. 2006). Thus, optimization strategies for seed train development are needed to achieve rapid, steady log phase growth without loss of downstream differentiation potential or viability.
Several strategies to reduce seed train times and save on costs have already been conceived of in other bioprocess applications and should be translatable to cultivated meat production. For instance, high density cryopreservation of cells (~10 x 10^7 cells/mL) can be thawed directly into multi-liter rocking platform bioreactors to dramatically reduce the number of days required to scale cell numbers, which in turn would save on costs (Tao et al. 2011). Alternatively, stem cells may be grown in a continuous or semi-continuous bioprocess early on, with subsets of cells from these bioreactors used to directly inoculate larger volume tanks. In these cases, coupled cell retention devices can enable continuous processing at high cell densities, saving on downtime between runs (Xiaoxia and Buser 2016; Fisher et al. 2019). A continuous bioprocess can also be approximated by operating multiple bioreactor lines in parallel and offset by differing harvesting times. Economic modeling of integrated continuous processing has shown savings of 55% on capital and operating expenses over a 10-year period [for production of monoclonal antibodies] compared to batch processing, suggesting that implementation of continuous processes would be attractive for cultivated meat companies (Walther et al. 2015). Some companies in the cultivated meat sector are now exclusively focused on developing continuous manufacturing technologies. For instance, CellulaREvolution has proposed using a novel peptide coating to permit cell attachment, growth, and detachment over time, with new immature cells taking the detached cells’ space, resulting in a continuous process.
Other strategies may be focused on growing higher densities of cells in lower unit volumes. Perfusion bioreactors such as hollow fiber reactors serve as one example to generate high-density cultures, capable of continuous cell growth over many months with high automation potential (discussed later) (William G. Whitford John J.S. Cadwell 2011; Allan et al., 2019). Cellular Agriculture Ltd is one example of a company developing a hollow fiber bioreactor tailored to cultivated meat-relevant cell types for scalable manufacturing. Additionally, new techniques utilizing co-extruded cells within 400 micron diameter alginate-based tubes (Lin et al. 2019; Li et al. 2018) offer tremendous potential for scaling cultivated meat production within closed microenvironments, bypassing seed train processing altogether and dramatically reducing the volume requirements of the bioreactor in order to obtain the same number of cells versus previously discussed methods. The range of densities achievable in different bioreactor systems can vary widely and may dictate the choice that companies take in their scale up. Table 1 gives some ranges and theoretical densities achievable for different bioreactor systems.
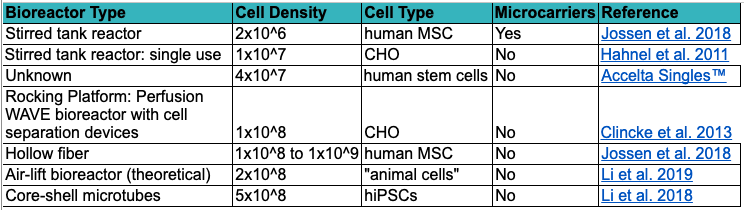
Table 1. A snapshot of recorded cell density ranges using different bioreactor types. This list is not intended to be exhaustive and achievable densities can change depending on cell type, species, and culture conditions.
Taken together, the bioprocessing scale-up strategy is one of the most substantial technical and engineering hurdles to overcome in order for cultivated meat production to reach mass markets. While it may take significant time to empirically validate or translate existing strategies in the biologics and cell therapy fields to cultivated meat production in new species and cell types, the tremendous evolution of bioprocessing over the last few decades provides a roadmap for success. Sharing of ideas and novel technologies across the biologics, cell therapy, bioreactor, and cultivated meat industries promises to provide positive synergistic outcomes across these fields.
Processing timelines
A general timeline for the production of cultivated meat is on the order of a handful of weeks, however, this can vary greatly based on the cell type, species, bioprocess design or culturing conditions, and downstream intended product. For instance, the developmental timelines of a species are generally recapitulated in vitro such that skeletal muscle derived from mouse pluripotent stem cells via directed differentiation can be produced in two weeks, whereas a human system will take three weeks or more (Chal and Pourquié 2017). Accordingly, the doubling times of the same cell types from different species can vary (Cheng et al. 2014). A summary of published studies found that mouse pluripotent stem cells grown in suspension cultures had doubling times of around 20 hours, whereas human pluripotent stem cells ranged from around 60-80 hours. By adjusting the cell culture media formulation of the human pluripotent stem cell to resemble a naive state, a term for a stem cell state similar to the preimplantation embryo and inherent to mouse pluripotent stem cells, human pluripotent cell doubling times decreased to around 30 hours (Lipsitz et al. 2019). Similarly, co-culturing of mouse embryonic stem cells with human embryonic stem cells resulted in acceleration of the developmental and differentiation timelines of the human cells (Brown et al. 2020). This suggests that the identification of secreted factors from mouse cells could be purified and used to accelerate processing timelines.
In another study, mouse embryonic stem cells grown on a feeder layer of embryonic fibroblasts displayed doubling times of 12-16 hours, whereas the same cell embryonic stem cell lines had doubling times of ~30 hours when grown in suspension (Tamm, Pijuan Galitó, and Annerén 2013). The doubling times of different cell types within a species can also vary. For instance, mouse adult myoblast cells display doubling times of ~24 hours (Shahini et al. 2018). Thus, the media and growth conditions can dramatically influence the observed doubling times of a cell line and large timeline efficiency gains to produce a certain number of cells may be granted solely on the selection of species, starting cell type, and manipulation of growth conditions. Optimizing doubling times or differentiation timelines can reduce the overall residence time in bioreactors, leading to reductions in cost and environmental footprint.
Other variables such as cell size in larger animals (Han et al. 2013) is also recapitulated in vitro, which may make up for slower doubling times in terms of gross tissue mass. Maturation time may also influence timelines and end products with different texture requirements. Thus, economic modeling of the bioprocess should take into account the different variables presented for each species throughout the bioprocess, many of which will need to be empirically determined due to a dearth of rigorous literature for many species relevant to cultivated meat.
Scale out vs. scale up
Given the challenges in scaling up cell production, a scaling out approach may yield a faster route to getting cultivated meat in the mouths of consumers. In this scenario, production takes place at smaller scales, satisfying meat production requirements that are more akin to a restaurant or butcher shop rather than a farm or a large slaughterhouse. These scales have been estimated to require more manageable bioreactor sizes of 100 – 1000L volumes to reach local demand. Thus, it may be significantly easier to design a scale-out facility with higher automation potential, lower energy, land, and capital expenditure requirements, and saved time and money from avoiding large scale up challenges. Similar strategies have been conceived at even smaller scales, with multiple groups developing in-home kits. Thus, scaling out approaches may provide a more reasonable short-term path to market for cultivated meat products and exploration of this strategy should be encouraged. However, production volumes at these scales will likely fail to meet the large demands for global meat consumption as they would not be able to capitalize at later stage exponential growth. Co-existing systems of local scale-out and large scale-up approaches may therefore be required to meet these demands over time.
Non-cellular considerations and sensor systems
The interactions amongst cells (solid phase) suspended in a liquid phase and aerated by a gas phase within a bioreactor are complex and require sophisticated monitoring to ensure a bioprocess is operating optimally. In general, these interaction variables can be broken into physical (e.g. temperature), chemical (e.g. pH), and biological (e.g. cell concentration). The sensors that measure these variables can lie inside the bioreactor itself (in-line), outside of the bioreactor (at-line or off-line), or operate continually (on-line) (Biechele et al. 2015). In-line sensors require enduring the same temperature and sterilization processes as the bioreactor itself, having minimal leaching of components, and potentially operating for weeks at a time without requiring re-calibration. If there are inhomogeneities within the bioreactor, usage of multiple in-line sensors or sensor arrays may be required to achieve accuracy. On the other hand, off-line sampling can require manual human involvement and sample manipulations, which can affect more time-sensitive variables and introduce contaminants (discussed later). In general, current sensor development focuses on real-time, on-line monitoring systems, which are frequently becoming enabled by advanced spectroscopic systems and a push for continuous bioprocessing in other industries. These sensors are primarily being developed to adhere to the FDA’s Process Analytical Technology guidelines but can be adopted by the cultivated meat industry. The key variables affecting a cultivated meat bioprocess are temperature, oxygen, carbon dioxide, pH, glucose, biomass, and metabolites. These variables and their sensing systems are discussed independently below.
The temperature of a cultivated meat bioprocess will depend on the species of interest. For example, most mammalian cells are grown around 37ºC while insects, fish, and other marine creature cells may be grown at significantly lower temperatures (Rubio et al. 2019, Rubio et al. 2019). Nevertheless, recording and maintaining accurate temperatures during the process is crucial for many parameters. This is typically done through the use of platinum resistance thermometers that contain a stable, non-reactive piece of platinum wire. The electrical resistance of the wire increases linearly with temperature and thus temperature can be inferred by passing current through the wire (O’Mara et al. 2018).
Oxygen is often the rate-limiting substrate within a bioreactor, as approximately 45 times the amount of dissolved oxygen can be carried by blood versus cell culture media (Martin and Vermette 2005). Oxygen must be continually delivered in the form of dissolved oxygen in order to meet cellular metabolic demands, referred to as the oxygen utilization rate. Meeting a specific cell line’s oxygen utilization rate requires considerations influencing the oxygen mass transfer coefficient, kLa, such as mixing speed, bubble size, temperature, flow rate, and general properties of the cell culture medium. Thus, species’ cells grown at lower temperatures, such as fish, may require differing oxygen requirements, as oxygen solubility increases at lower temperatures. Without proper oxygenation (typically 30-40% of air saturation) or with over-oxygenation, cell growth and viability can be rapidly negatively affected. Several methods exist for monitoring liquid phase dissolved oxygen, however membrane-covered amperometric electrodes, optical sensors, and paramagnetic sensors are most practical for biological applications (Biechele et al. 2015; O’Mara et al. 2018).
Carbon dioxide and pH are intimately linked in a cultivated meat bioprocess. As a bioprocess scales, an increasing number and density of cells respire, leading to an increasing concentration of dissolved CO2 above the standard 5-10% operating range. The CO2 can readily diffuse across the cell membrane, lowering intracellular pH and affecting cellular metabolism, enzyme function, protein folding, and general cell health (Pattison et al. 2000). In order to decrease CO2 concentrations, surface aeration, sparging, or agitation can be used; however, each becomes more difficult with scale due to surface-to-volume limitations and shear stress considerations (Matsunaga et al. 2009). CO2 is typically measured using a Severinghaus electrode, which contains a CO2-permeable membrane and electrode that records the resulting pH change within a bicarbonate solution as CO2 is absorbed. pH indicators can also be used to adapt this methodology to optical sensing methods (Ali et al. 2010).
The pH of the cell culture medium must be tightly controlled for optimal cell health (for mammalian cells, generally 7.4 ± 0.4). Together with oxygen, the pH can yield information about cell growth rate and metabolism, as acidic byproducts of cellular metabolism such as lactic acid and carbonic acid (following CO2 reacting with water) accumulate in the medium, resulting in lower pH. pH is typically monitored via potentiometric and optical sensors. Indeed, the standard red-pink color associated with cell culture is caused by the dissolved pH indicator phenol-red, which adjusts color under certain pH thresholds (in this case, your eye is the optical sensor). Similar indicators can be used in immobilized substrates attached to optical fibers for more practical pH monitoring. The limitations of optical methods include a limited dynamic range, heat instability of some fluorophores, and sensitivity to mediums with high ionic strength (Biechele et al. 2015). Lastly, ion-sensitive field effect transistors can record pH by measuring current changes caused by ion (H+) concentration changes; however, these devices require parallel reference electrodes.
Glucose acts as the principal carbon energy source within a cultivated meat culture system and thus active monitoring of glucose and other metabolites can inform feeding strategy, optimal cell densities, and proliferative rates. As glucose and glutamine are consumed during exponential cell growth, lactate and ammonium, respectively, increase in the medium, which can have prohibitive effects on cell growth and viability. Several methods aimed at decreasing or controlling these byproducts have been developed (Freund and Croughan 2018), although ammonium toxicity is far more potent (2-4 mM) than lactate (20-40 mM) and thus can be a more important target to reduce (Schneider, Marison, and von Stockar 1996). Glucose and a range of metabolites are typically measured via spectroscopic analyses (Figure 11), although some enzymatic methods also exist. The methods primarily used in detecting biological molecules such as infrared, fluorescence, and Raman spectroscopy fundamentally rely on the interaction of electromagnetic waves with molecular bonds which reveal chemical fingerprints based on measured vibrational motion (infrared), scattering of vibrations (Raman), or emitted photons upon excitation (fluorescence). These sensor systems are advantageous as they can be used continually, there is no interaction between the sensor and analyte itself, and they can be multiplexed.
Infrared spectroscopy includes the near- (740-1300 nm) and mid- (1300-15000 nm) infrared wavelengths. Each can detect relevant organic molecules such as glucose and lactate, but certain wavelengths are more useful for molecules containing specific chemical bonding structures (Biechele et al. 2015). Using mid-infrared wavelengths can yield stronger signals and higher resolution for these purposes. Raman spectroscopy relies on electromagnetic waves typically in the visible or near infrared range and can complement infrared methods. In general, implementation of spectroscopic methods can be challenging due to relatively low concentrations of the molecules of interest, the diversity of molecules present, difficulty in calibration due to changing conditions within a live cell culture, and the large amount of data that is generated (Zhao et al. 2015). Therefore, large sets of training data from pilot or spike-in studies may be required in order to apply chemometric statistical techniques that permit identification of individual molecules of interest with high confidence. Data-driven models from chemometric analyses can then feed into a soft sensor (a sensor combined with a software-based algorithm) algorithm for a custom cell-based meat bioprocess.
Similar spectroscopic methods can also be used to detect biomass concentration, including bacterial contaminants, based on scattering or transmission of light through a turbid sample. Near-infrared wavelengths are typically used for larger mammalian cells, with specific wavelengths between 840 and 910 nm as typical culture mediums do not absorb much light in these wavelengths (Ahmed et al. 2014). However, these methods make no determination of cell viability and can be affected by air bubbles or other large particles within the cell culture. These issues can be partially alleviated through the use of impedance sensors where measurement of a sinusoidal electrical field between two electrodes is minimally affected by objects such as air bubbles with no plasma membrane. Impedance methods can be adapted for measurement of cell densities within a solid scaffold, where spectroscopy may otherwise fail. Lastly, in situ microscopy and flow cytometry can also be incorporated into a bioprocessing scheme if additional morphological, size, or viability data are needed (Biechele et al. 2015), although the practicality of implementing these methods for cultivated meat may be limited.
Recent advances in genetic engineering permit the design of genetically-encoded biosensors that can sense extrinsic (e.g. shear stress) or intrinsic (e.g. oxidative damage) characteristics of cells (Polizzi and Kontoravdi 2015). Fundamentally, genetically encoded sensors rely on the transcription of specific genes tied to a specific characteristic. Using these gene promoters to drive expression of a detectable reporter protein such as green fluorescent protein (GFP) or product of an enzymatic reaction (e.g. light from luciferase) can provide critical insight into a cell population’s interaction with its environment. Some examples include genes involved in the unfolded protein response to sense glucose or amino acid starvation (Kaufman et al. 2002), hypoxia response genes (Liu et al. 2005), mechanical stress genes, and protein production (i.e. endoplasmic reticulum stress response) genes (Du et al. 2013). Building biosensors into an optimized cell line may offer several advantages over the aforementioned methods, including savings on cost, calibration, sterilization, time sensitivity, and flexibility. However, inhibitory metabolic burden from a constitutively active biosensor would need to be considered. Whether or not a product can be consumed with these transgenes in them is yet to be determined by regulation. However, receiving Generally Recognized as Safe (GRAS) designation by the FDA may not be insurmountable, as many of these biosensors are derived from animal proteins (e.g. GFP from A. victoria and luciferase from P. pyralis) rather than being purely synthetic.
Automation
Incorporating automation into a bioprocess can unlock significant cost savings on manual labor time, reagent use, lab space, and general gains from limiting batch-to-batch variation and standardizing quality control. Automation can also facilitate the prevention of contamination and ease regulatory compliance when implemented in a closed system. Automation is just starting to gain traction in the cell therapy and regenerative medicine fields. Several products now exist that enable closed, end-to-end manufacturing of therapeutic doses of adipose-derived stem cells from adipose tissue biopsies (Fraser et al. 2014) or general stem cell expansion with high degree of customization and parallelization. Despite these advances, cultivated meat manufacturing fundamentally involves orders of magnitude higher numbers of cells and thus may require significant engineering customization, especially in regards to cell scaling, quality control, harvesting, and product formulation. These customizations may require considerable capital expenditure and may therefore be better suited to be built out as hardware or software offerings from existing life science companies and technology stakeholders rather than internally within a startup. Applying learnings from the existing lower volume bioprocess industry and the larger volume food and agtech industries, where automation is abundant, may be favorable for implementation in cultivated meat production.
In general, automation should be designed to be implemented into the manufacturing process from the ground up, rather than retroactively replacing highly manual steps (Ball et al. 2018). While species or product specificity may dictate a custom implementation, the general bioprocess as described throughout this series is likely to be conserved. Thus, industry-wide collaboration toward identification of critical process parameters and the composition of their analytical frameworks to guide automation implementation would pay dividends for the entire industry. Similarly, these parameters may guide industry standardization across software (open-source), sensor systems or other hardware components, and safety and regulatory considerations, although sacrificing the potential for intellectual property development would need to be considered. Collaborative efforts are also needed to establish cost of goods (COGS) models for cultivated meat from which high-cost areas or bottlenecks within the bioprocess can be identified and prioritized for automation or engineering optimization. The nascent nature of the industry may reveal that some critical process parameters need to be better defined, however eventual automation throughout the industry is likely to be pursued to achieve price parity with conventional animal meats.
Sterilization
While an academic cell culture lab may involve several individuals opening and closing an incubator door and walking freely through open air with containers of cells, an industrialized bioprocess is largely sealed off from the outside world. Notwithstanding previously discussed single-use bioreactor systems, a large effort has been dedicated to developing preventative controls and contamination monitoring methods so as to not incur losses in product, production time, or cost of decontamination, and to ensure adherence to regulatory and safety guidelines in other biological processing industries. Many of these controls and best practices should be translatable to the cultivated meat industry.
The most common forms of contamination are from adventitious agents such as bacteria (e.g. mycoplasma), fungi, viruses, or from cell cross-contamination, with some concern for transmissible spongiform encephalopathies (Piccardo et al. 2011). It should also be known that any cell lines that are banked will have already been previously screened for contamination of adventitious agents prior to being cryopreserved, as is standard in industry. Thus, prevention of adventitious agents from entering bioreactors during the bioprocess and having robust monitoring systems will ensure product safety.
As discussed previously, spectroscopic sensors to detect bacterial contamination may be utilized, as well as other methods such as polymerase chain reaction or immuno-based assays (e.g. ELISA) for detection of specific contaminants or foreign adventitious agents. While sterility is ideal, it is possible that acceptable non-adverse contamination (e.g. tolerably low levels or non-pathogenic in nature) occurs, and there is always a risk for contamination events. Although antibiotics and antimycotics can be used for both treatment and prevention, they can lead to variations in gene expression (Ryu et al. 2017), proliferation (Figure 2; Kolkmann et al. 2020, Cohen et al. 2006), and differentiation (Y. Chang, Goldberg, and Caplan 2006). The presence of antibiotics has been shown to significantly reduce bovine Most importantly, proper practice of aseptic technique, implementation of preventative controls (discussed below), and sterilization strategies make antibiotic and/or antimycotic use in cell culture mediums unnecessary. As such, they will not be included in a cultivated meat bioprocess. It is possible that antimicrobials may be used in maintaining aseptic surfaces.
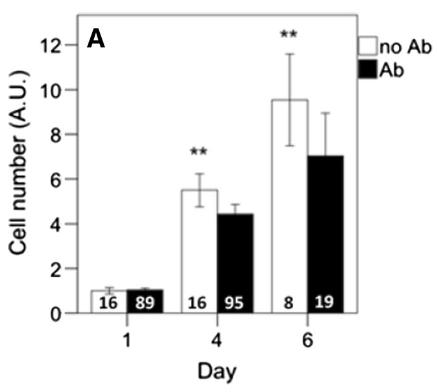
Figure 2. Primary bovine myoblasts proliferate significantly less in the presence of antibiotics. From Kolkmann et al. 2020.
Different components of the bioreactor are susceptible to entry of various contaminants and thus require preventative barriers to entry, which maintain the sterile boundary. For gas or medium inlets and outlets, various membranes or filters can be used to capture potential contaminants via size exclusion. For instance, a 0.22 or 0.1 µm pore size filter permits steady flow of gases and fluids but will prevent passage of cells as small as bacteria. Smaller pore size filters (e.g. 20 nm) also exist for viral retention. Filters for medium are used primarily when steam sterilization (discussed below) may potentially damage heat-sensitive ingredients in the medium. Gas filters are commonly made of hydrophobic membranes such as PTFE to prevent passage of aqueous aerosols. These filters and other connective parts are commonly made of materials that can themselves be sterilized via gamma irradiation or autoclaving to ensure they are free of contaminants upon installation. Other forms of sterilization used in the food industry such as pulsed electric fields, which cause cellular permeabilization, may be adapted for prevention of bacteria and bacterial spore contamination of cell culture media (Reineke et al. 2015). Additionally, high temperature short time sterilization also known as flash pasteurization can be used to inactivate viruses but similarly poses some threat to heat-sensitive ingredients.
In addition to filtering and radiation-based sterilization strategies, thermal sterilization via steam is the most commonly used method in biopharma with stainless steel stirred tank reactors. Thermal sterilization is often performed after cleaning of vessels or process components, adhering to clean-in-place guidelines where equipment is washed with high-pressure water jets, rinsed in alkaline and acidic solutions, and dried. Steam sterilization can be performed directly at inlets and outlets, in an empty vessel, on medium within a vessel, or on medium flowing through a continuous process line. When performed both upstream and downstream throughout an entire bioprocessing line, the act is referred to as steam-in-place. Steam sterilization parameters, estimated by measuring the death kinetics of the heat resistant bacteria B. stearothermophilus, are dependent on many variables including time, temperature, moisture (i.e. saturated steam), direct steam contact, air removal, and drying. Differences in the heating and cooling requirements and times can vary depending on the scale and other process variables. Due to the high temperatures required (usually ≥121ºC), these variables can influence the total energy demand of a cultivated meat facility at scale, affecting its carbon footprint. As such, alternative bioreactor types may be pursued to, in part, avoid these negative externalities. Once sterilization has been achieved, the bioreactors operate under positive pressure, typically in the form of an inert nitrogen gas, in order to prevent entry of contaminants. If contamination is detected, investigation into the source is performed (e.g. faulty filters, micro-cracks, improper sealing) and components are cleaned and re-sterilized.
View references featured in bioprocess design
Header image courtesy of JUST.