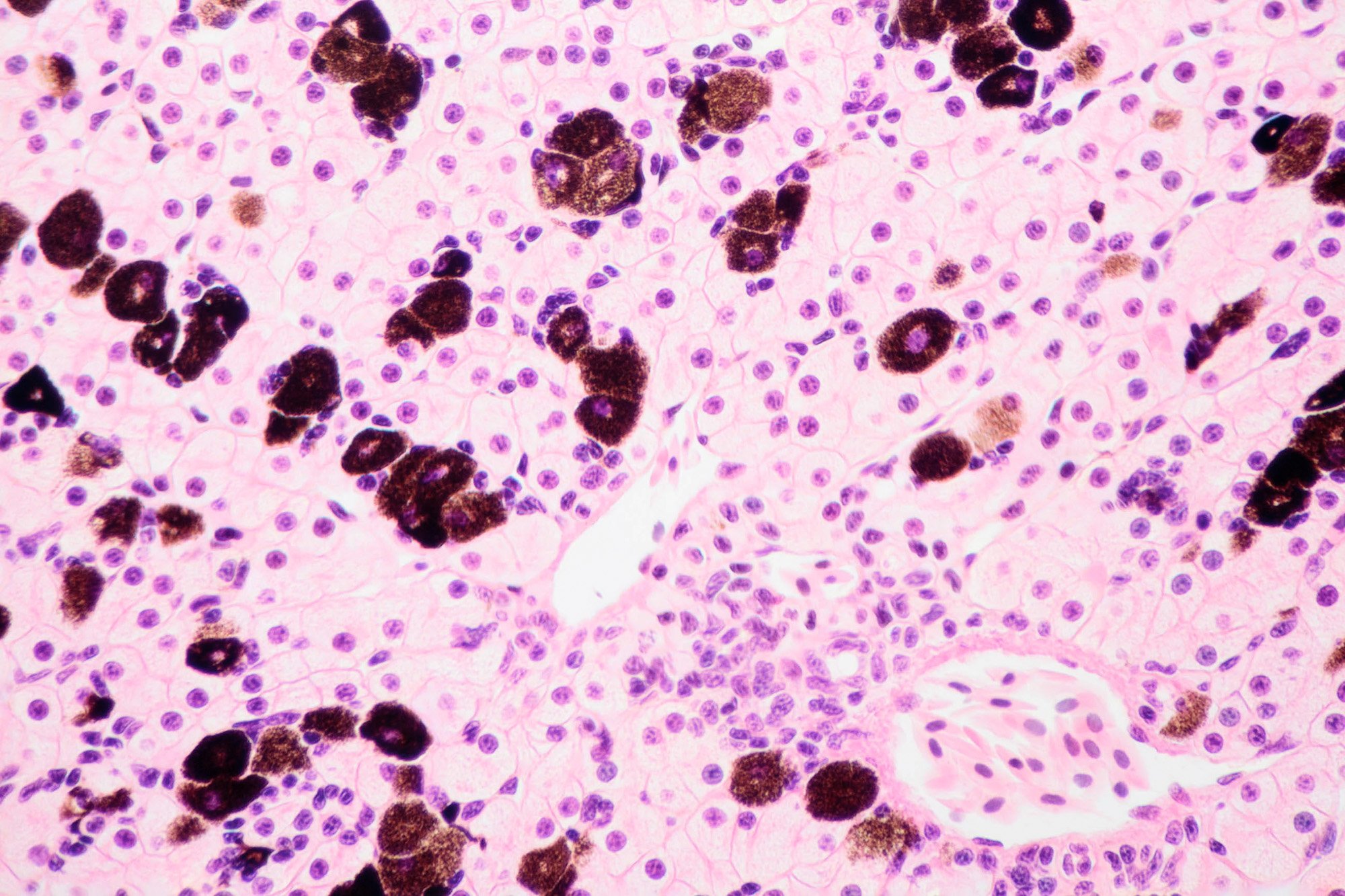
Deep dive: Cultivated meat cell lines
Learn about the cell lines that are important for cultivated meat production.
Cell lines
Introduction
The cell lines used in cultivated meat production ultimately determine many of the downstream variables to consider. As starting material, cells that can self-renew and differentiate into the cell types that make up meat tissue (i.e., myocytes, adipocytes, fibroblasts, chondrocytes, endothelial cells, etc) are most attractive (Lee et al. 2023; Jara et al. 2023; Reiss et al. 2021).
Several different possibilities exist for the starting cell population, delineated by their potency, or ability to differentiate into a diversity of cell types. For instance, embryonic stem cells have the ability to differentiate into cells of all three developmental germ layers (i.e., ectoderm, mesoderm, and endoderm), while adult stem cell populations found throughout our body, such as mesenchymal stem cells (MSCs) and muscle satellite cells, are typically more specialized and limited in their potency to differentiate into cells of the same germ layer or organ type. The balancing act between potency and differentiation has important implications to the overall cultivated meat production process (Figure 1A). Highly potent cells, such as embryonic and induced pluripotent stem cells, have desirable traits such as rapid division and retained immortality. However, their ability to differentiate into any cell type means that extra effort needs to be dedicated to coaxing the cells to differentiate into a single desirable lineage (e.g., skeletal muscle or fat). On the other hand, adult stem cells, which are not inherently immortal and may divide more slowly, require less effort to differentiate into their designated lineages. Manufacturers that use pluripotent stem cells may therefore employ different media formulations, process strategies, and production time lengths (discussed throughout other deep dives) compared to those that use adult stem cells.
The cultivated meat industry has not yet consolidated around a particular cell type as the optimal starting point. It remains to be seen whether this will happen as the industry matures or whether different starting cell types will be preferred depending on various aspects of the bioprocess or the desired end product. The most likely starting cell types for use in cultivated meat production and the main targets for differentiated cells are shown in Figure 1B-C and discussed below.
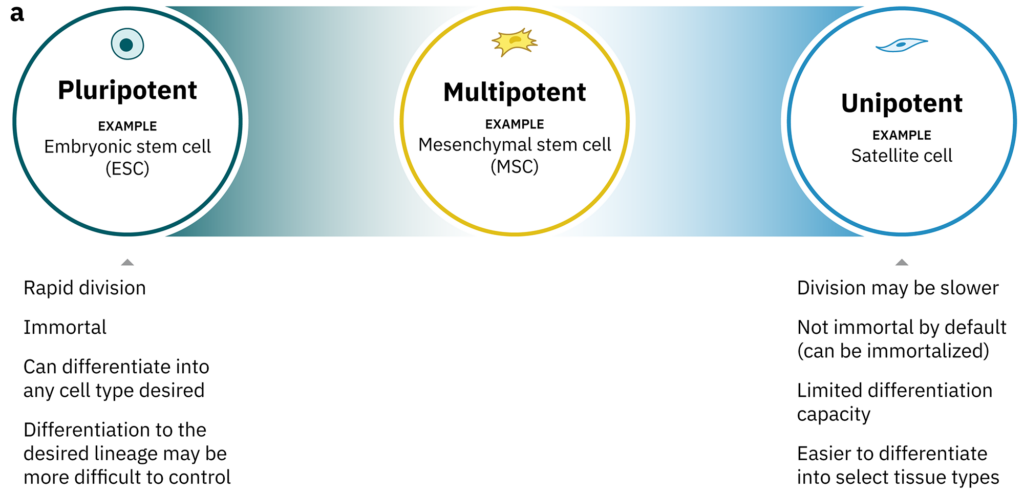
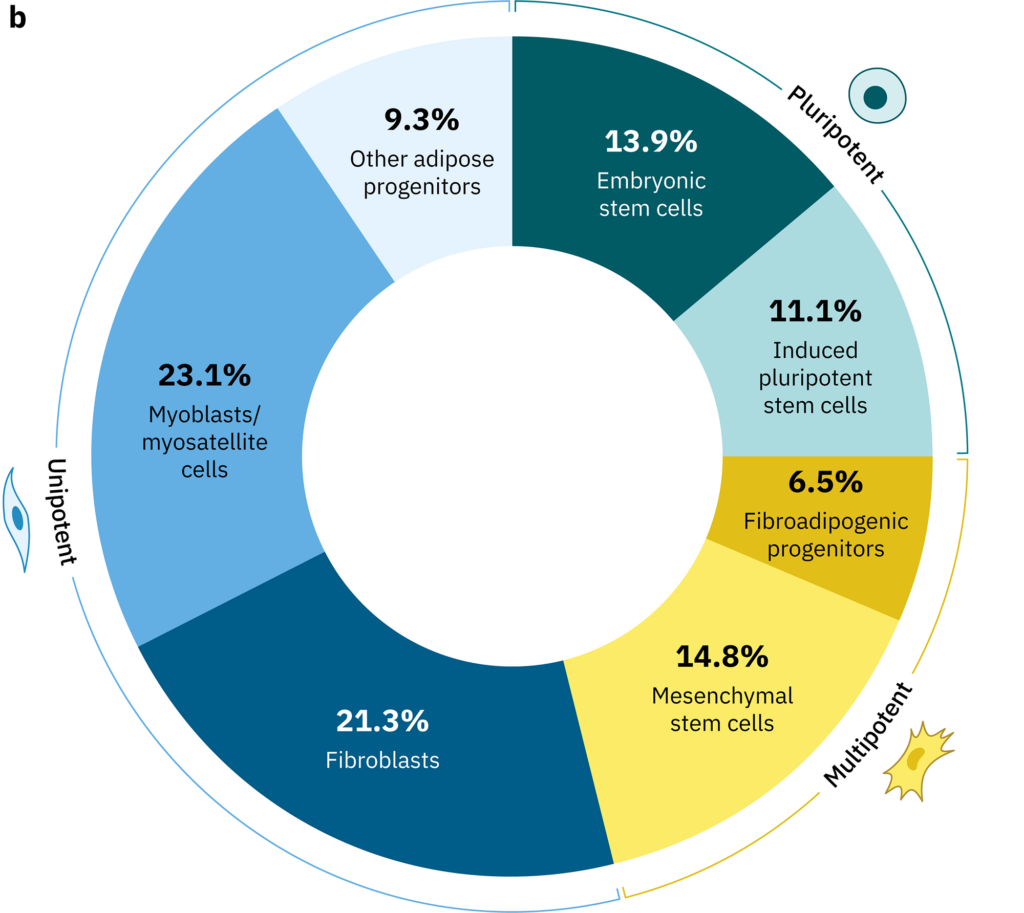
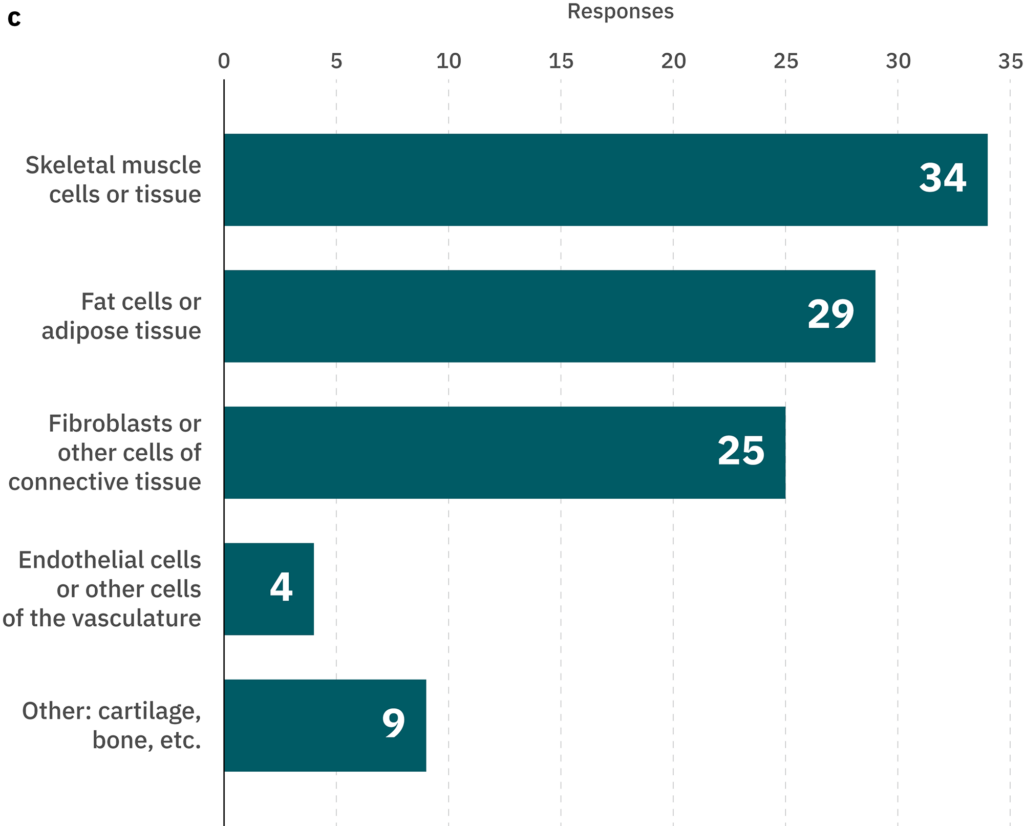
Figure 1. (A) Trade-offs involved in the decision to use pluripotent or unipotent cell types. Specific cell types shown are examples of pluripotent, multipotent, and unipotent types that might be used in cultivated meat production. Some advantages and disadvantages of pluripotent types are listed. Multipotent cell types such as MSCs may represent a compromise between the two extremes. (B-C) Results from a survey of cultivated meat producers. The survey questions were (B) The principal cell type(s) my company currently works with is/are _____ (select up to four)” (no. of respondents = 40) and (C) “At my company, we produce or plan to produce _____ in the next 12 months (select all that apply)” (no. of respondents = 40). Outer labels in (B) indicate the potency of the indicated cell types, though please note that these are general categories only and may not hold true in every case, e.g., some types of fibroblasts and adipose progenitors could be classified as multipotent rather than unipotent.
While not discussed throughout, it is also possible to use primary cell lines from specific organs or tissues for the generation of other consumable products, such as foie gras derived from hepatocytes or other organ tissues such as fish maw (swim bladder). The growth of mammary epithelial cells that create the nutritional components of milk (i.e. lactate, casein, and whey) can also be used to create human or animal milk products. Non-meat products fall under a larger umbrella of cellular agriculture, which include agricultural products such as wood, leather, enzymes, animal proteins, and other materials produced using animal, plant, or microbial cell cultures.
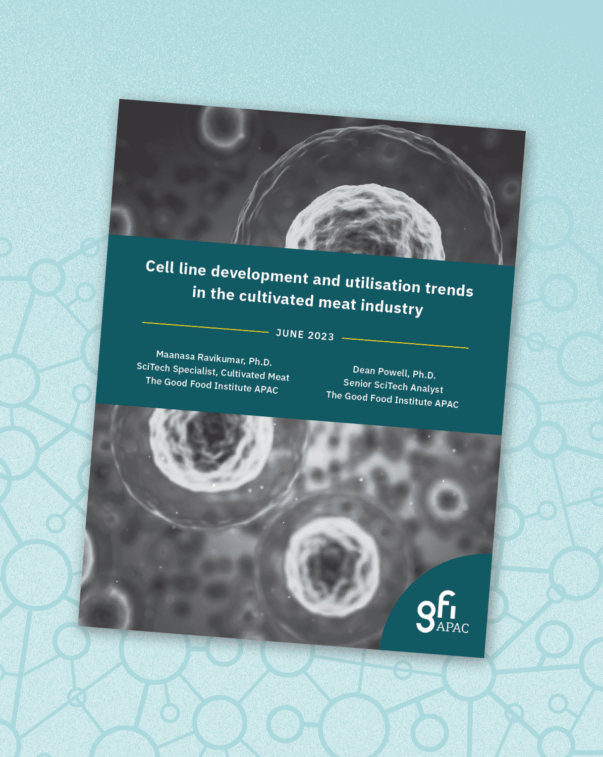
Cell line development and utilisation trends in the cultivated meat industry
Cell lines are the foundational building blocks that make cultivated meat production possible. However, an unavailability of cell lines with desirable characteristics for commercial production is a major bottleneck across the industry. To untangle the complicated web of challenges that cultivated meat manufacturers and cell line providers are encountering, GFI APAC sent a questionnaire to a long list of startups and companies at the end of 2022. A total of 44 companies responded to the survey, providing a first-of-its-kind portrait of the progress, preferences, and hurdles at play in this fast-growing global industry as it seeks to diversify how meat is made.
Pluripotent stem cells
To use embryonic stem cells (ESCs) as a source for cultivated meat production, one must first have access to an embryonic stem cell line. ESCs are derived from the inner cell mass of an early embryonic structure called a blastocyst, which forms just a few days post-fertilization. The first embryonic stem cell lines were derived from mice in 1981 (Evans and Kaufman 1981; Martin 1981) and were followed thereafter by a select few additional species including human, non-human primates, rat, chicken (Pain et al. 1996; Petitte et al. 2004), and fish. However, derivation of stable embryonic stem cell lines is extremely challenging, as the embryonic material is difficult to obtain and work with, the cells are highly sensitive to their growth substrate, and they require different sets of growth factors or inhibitors from species to species to maintain proliferation without spontaneous differentiation.
Indeed, the derivation of embryonic stem cell lines from agriculturally-relevant bovine species was only recently achieved in 2018 (Bogliotti et al. 2018). Subsequently, simplified culture conditions for the derivation and maintenance of bovine ESCs enabled the derivation of 10 independent lines (Soto et al. 2021). Bovine ESCs have also been reported by Kinoshita et al. (2021) (Figure 2). Beyond the simple requirement for pluripotent lines to exist, the availability of straightforward and cost-effective protocols for their derivation opens up new avenues for the derivation of lines from multiple individuals and breeds, and the subsequent selection of lines that maximize both bioprocess efficiency and flavor.
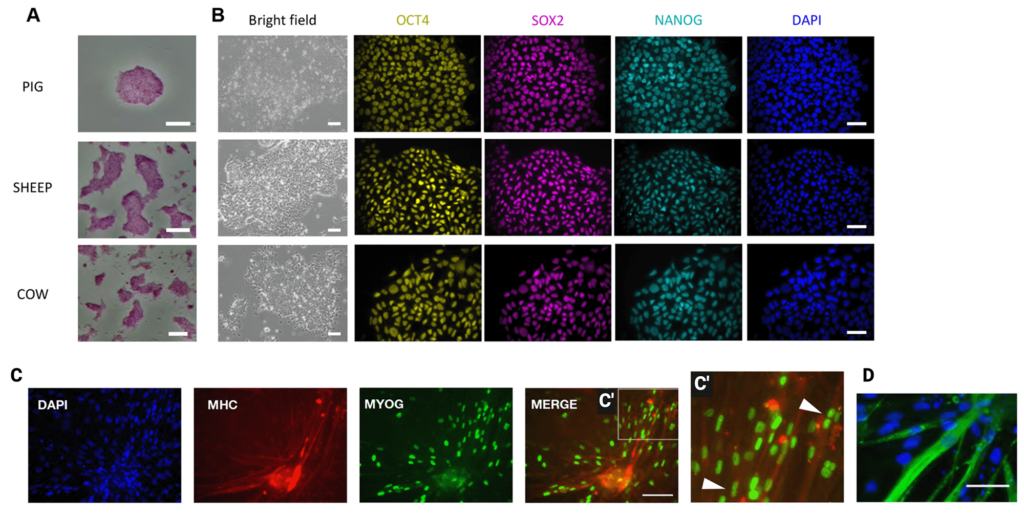
Figure 2. Establishment of pluripotent stem cells from livestock mammals. (A) Alkaline phosphatase (AP) staining of cell colonies. Passage numbers are: 17 (pig), 20 (sheep) and 12 (cow). AP staining (A) and immunofluorescence of OCT4, SOX2 and NANOG (B) was performed on at least three lines from each species. (B) Bright-field images of embryonic disk stem cells (EDSCs) from pig (P9), sheep (P8) and cow (P21) embryos. Scale bars: 100 μm. Colonies were stained for OCT4, SOX2 and NANOG, and with DAPI. (C) Immunofluorescence staining of MYOG and myosin heavy chain (MHC) in differentiated bovine EDSCs. C′ is a higher magnification of the boxed area with arrowheads indicating multiple nuclei in MHC-positive cells. Scale bar: 100 μm. (D) Differentiated bovine cells immunostained for the striated muscle marker TITIN (green) and stained for DAPI (dark blue). Scale bar: 25 μm. Adapted from Kinoshita et al. (2021) under the terms of the CC BY license.
The generation of ESCs from pigs has historically proven similarly challenging (Zhang et al. 2022), though recent studies have been more successful (Gao et al. 2019; Choi et al. 2019; Kinoshita et al. 2021, Figure 2). In 2022, pluripotent stem cells were derived from the pig epiblast, and these same cells were later used to create a cultivated pork prototype (Zhi et al. 2022; Zhu et al. 2023). While the distinction between stem cells derived from the inner cell mass (i.e., embryonic stem cells) and those derived from the epiblast is certainly consequential for some applications, epiblast-derived cells are capable of differentiating into all of the cell types relevant to cultivated meat, and are therefore probably just as viable a source of starting cells.
A stable ESC line was also recently derived from sheep blastocysts (Vilarino et al. 2020). Consistent with the idea that cell isolation and maintenance protocols will need to be optimized for individual species, the same study found that many of the isolation conditions tested—which had previously been shown to be successful in humans and mice—were unsuccessful in sheep. Sheep ESCs were also recently reported by Kinoshita et al. (2021, Figure 2).
While access to appropriate cell lines for cultivated meat remains a challenge, availability is steadily improving. As of late 2023, ESCs or ESC-like cells from European seabass, zebrafish, cow, pig, and sheep can be purchased or licensed from various sources (see list at the end of this section). If cultivated meat is to be available for every commonly-eaten animal species, similar efforts will be necessary to expand the pool of available pluripotent cell lines to include other species of culinary relevance.
As an alternative to ESCs, scientists can utilize a technology called cellular reprogramming to obtain induced pluripotent stem cells (iPSCs) that maintain the desirable properties of ESCs without being derived from an embryo. Cellular reprogramming enables the direct conversion of one cell type into any other cell type based on the expression of a defined set of important genes of the final cell type (Rackham et al. 2016), typically a set of transcription factors. Reprogramming can be performed via viral-mediated over-expression of transcription factors, either via permanent genome integration (e.g., through the use of lentivirus) or stochastic non-integrating expression (e.g., via Sendai Virus (Fujie et al. 2014)) based on the virus type. Alternatively, reprogramming can be achieved by non-integrating methods such as episomal or mRNA gene delivery (Schlaeger et al. 2015), proteins (Cho et al. 2010), or small molecules (Zhang et al. 2012). Non-integrating methods for reprogramming are likely to be preferred from a regulatory, food safety, and consumer acceptance perspective.
The generation of iPSCs can be performed from virtually any adult somatic cell, including easily obtained cells such as white blood cells or skin fibroblasts, by over-expressing the canonical set of transcription factor genes Oct4, Klf4, c-Myc, and Sox2, referred to as Yamanaka factors (Takahashi and Yamanaka 2006). Because these genes are highly conserved, iPSCs have been generated from many agriculturally-relevant species, including chicken (Rosselló et al. 2013), cow (Bressan et al. 2020; Bessi et al. 2021; Zhao et al. 2021; Su et al. 2021; Xiang et al. 2021), pig (Xu et al. 2019; Yoshimatsu et al. 2021), horse (Bressan et al. 2020), and rabbit (Honda et al. 2010). Examples of iPSC derivation from fish and other marine species are sparse and currently limited to zebrafish (Rosselló et al. 2013; Peng et al. 2019) and carp (Xu et al. 2021). iPSCs are typically easier to derive than ESCs while being generally equivalent in their functionality (Choi et al. 2015). Furthermore, the underlying principles of reprogramming make it possible to directly transdifferentiate starting cell types (e.g., fibroblasts) directly to other adult cell types, bypassing the iPSC state altogether.
ESC or iPSC lines have been described from a fairly wide variety of food-relevant species, including cow, pig, goat, sheep, Javan banteng (a species of cattle), water buffalo, horse, rabbit, chicken, quail, ptarmigan, sea bream, sea perch, cod, sea bass, turbot, and carp (Lázaro et al. 2024). Future research should focus on characterizing the suitability of these cell lines for cultivated meat production and developing efficient, food-safe, and scalable methods for promoting proliferation and differentiation.
Explore known pluripotent or embryonic cells
The table below includes pluripotent or embryo-derived cell lines that are likely to be useful in cultivated meat or seafood research. Some lines may be available through publicly-accessible repositories or by contacting the cell line owner directly. You can use the “Filter” button to narrow down the list based on species, cell type, availability, or other criteria.
Adult stem cells
Mesenchymal stem cells
Many tissues in the adult body contain a reservoir of stem cells needed to replenish cell populations due to injury, cell death, or normal cellular turnover. These are referred to as adult stem cells. One of the most studied adult stem cell types is the mesenchymal stem cell (MSC), sometimes also referred to as mesenchymal stromal cells. MSCs are most commonly obtained from purified cell populations originating from a bone marrow or adipose tissue biopsy, although other sources such as the placenta, dental pulp, or umbilical cord have also been cited (Pittenger et al. 2019). Indeed, the diverse set of tissue sources and resultant cell phenotypes has called into question how MSCs should be defined and whether they are stem cells at all (Sipp, Robey, and Turner 2018; Caplan 2017). While guidelines from the International Society for Cellular Therapy (Dominici et al. 2006) have aimed to better define MSCs, some criteria such as cell surface marker expression may not be applicable in species outside of humans and thus will need to be better defined for cultivated meat.
MSCs are typically defined in part by their ability to form osteoblasts, adipocytes, and chondrocytes, while MSC potency toward skeletal muscle cells is somewhat limited and can be dependent on tissue source. Cells isolated from bovine muscle tissue according to their expression of the β1 integrin receptor CD29 (a known MSC marker (Knežić et al. 2022)) were shown to differentiate into both myogenic and adipogenic lineages and to self-assemble into small, 3D “meat buds” (Naraoka et al. 2021). In addition to the tissue source, the isolation protocol matters. For example, the combination of physical (i.e., shaking) and enzymatic dissociation produced substantially higher numbers of bovine adipose-derived MSCs as compared to a protocol using enzymatic dissociation alone (Lee et al. 2023). In general, the multipotent nature of MSCs can be harnessed by culturing cells in specific cell culture medium formulations in order to bias their differentiation pathways. Thus, MSCs can serve as a readily attainable source of starting cells capable of making the principal cellular components of meat. MSCs have been isolated from chickens (Adhikari et al. 2018) and fish (Salmerón et al. 2016), and should—in theory—offer a promising source of starter cells for cultivated meat (Svoradova et al. 2021; Bomkamp et al. 2023). However, to our knowledge their use in this context has not yet been reported in the literature. Further research is needed to better define the differentiation potential of various classes of MSCs, especially with regard to adipogenic and myogenic lineages, and to develop and bank immortalized MSC lines for use in cultivated meat research.
Fibroblasts and myofibroblasts
Homeostasis of the skeletal muscle involves a variety of resident muscle cell types. Deposition of extracellular matrix components that make up the muscle connective tissue is a key feature in guiding homeostasis, repair, and regeneration. This extracellular matrix deposition can occur in muscle from cell types including fibroblasts, myofibroblasts, and fibroadipogenic progenitor cells. Given that the defining features of these fibroblast populations are still being delineated, it’s possible that those working with MSC populations derived from the skeletal muscle tissue will actually be culturing, in part, heterogeneous fibroblast populations (Fan et al. 2022).
Fibroblasts and closely-related cell types may play a role in cultivated meat production by secreting extracellular matrix proteins (Chong et al. 2022), thereby influencing the textural properties of cultivated meat products and possibly the behavior of other cell types during the culture period. In comparison to other cell types used as sources for cultivated meat, fibroblasts have the advantage of being easy to culture, with a wider variety of commercially-available lines. Because of this, transdifferentiation of fibroblasts and similar cell types into myogenic-like or adipogenic-like cell phenotypes may be a worthwhile strategy (Tsuruwaka and Shimada 2022; Tsuruwaka et al. 2015). The use of fibroblasts is discussed in the safety dossiers submitted to the relevant regulatory bodies by the cultivated meat companies UPSIDE Foods (Schulze 2022), GOOD Meat (Licari 2022), and Vow (Food Standards Australia New Zealand 2023), and is also under investigation by Believer Meats (Pasitka et al. 2022).
Explore known fibroblast and myofibroblast lines
The table below includes fibroblast and myofibroblast cell lines that are likely to be useful in cultivated meat or seafood research. Some lines may be available through publicly-accessible repositories or by contacting the cell line owner directly. You can use the “Filter” button to narrow down the list based on species, cell type, availability, or other criteria.
Preadipocytes and fibroadipogenic progenitor cells
Fibroadipogenic progenitor (FAP) cells are a special type of mesenchymal progenitor cell capable of differentiating into myofibroblasts, adipocytes, chondrocytes, or osteogenic cells, but not myoblasts (Biferali et al. 2019). Although not responsible for the production of skeletal myotubes themselves, FAPs can secrete a variety of growth factors and cytokines that increase or influence myogenesis and myogenic differentiation of myoblasts (Wosczyna and Rando 2018). These cell types are also known to influence the accumulation of fibroblasts and adipocytes within the connective tissue, especially in disease contexts. Committed but undifferentiated fat cells are often referred to as preadipocytes.
In the context of cultivated meat, FAPs or preadipocytes may serve as a cell source for cultivated fat. For example, Dohmen et al. (2022) demonstrated that bovine FAPs could be used to produce cultivated fat with a fatty acid profile similar to bovine subcutaneous fat (Figure 3). In addition, several other recent studies have described the use of adipogenic cells in cultivated fat production (Liu et al. 2023; Yuen et al. 2023; Louis et al. 2023).
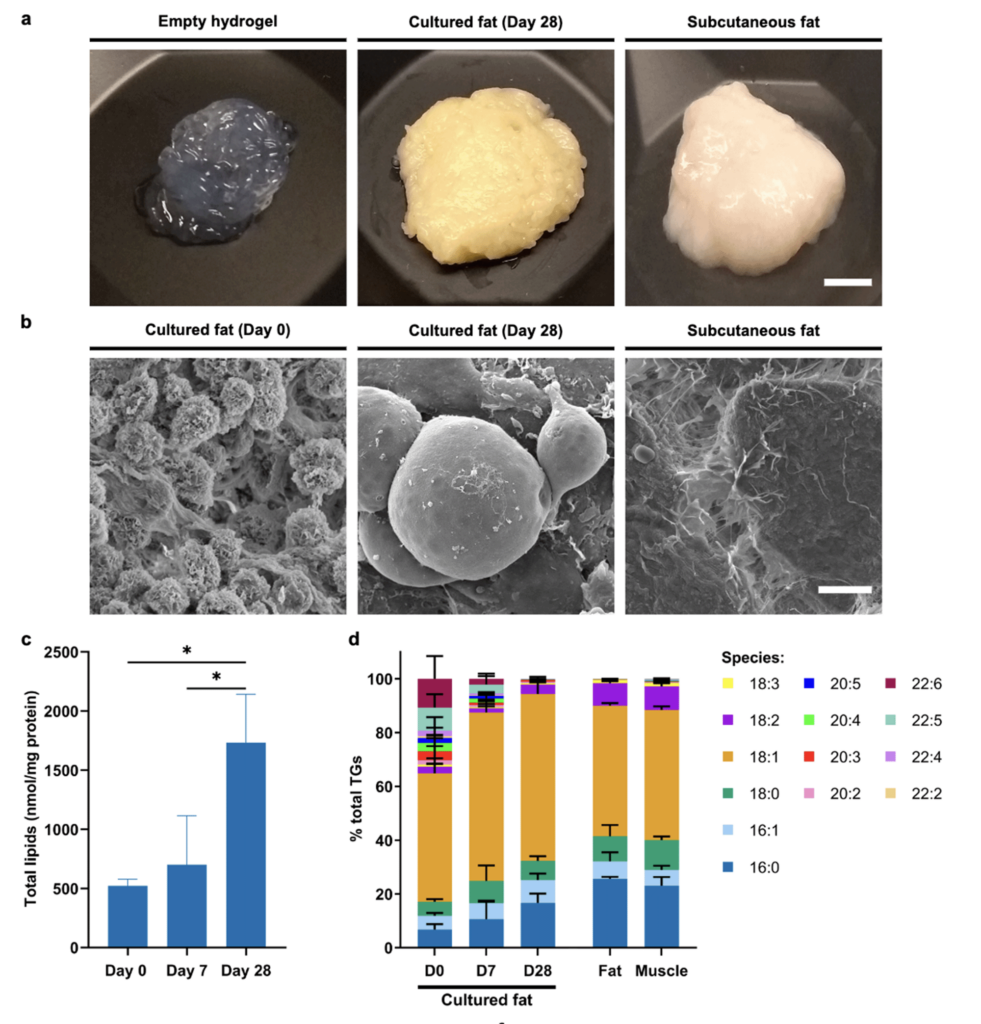
Figure 3. Lipidomic analysis of FAP-derived cultured fat. (A) Macroscopic photographs of empty alginate hydrogel, cultured fat (after 28 days of differentiation), and bovine subcutaneous fat. Scale bar = 5 mm. (B) Scanning electron microscope images of cultured fat after 0 and 28 days of differentiation, and bovine subcutaneous fat. Scale bar = 10μm. (C) Absolute quantification of total lipid content (normalised to mass of protein) of cultured fat after 0, 7, and 28 days of differentiation. (D) Breakdown of relative percentages of fatty acid species within the triglyceride lipid class in cultured fat samples after 0, 7, and 28 days of differentiation, and bovine subcutaneous fat (‘Fat’) and skeletal muscle (‘Muscle’) control samples. Legend denotes C:D numerical annotation (where C indicates number of carbon atoms, and D number of double bonds, in the acyl chain). Reproduced from Dohmen et al. (2022) under the terms of the CC BY license.
For cultivated meat to live up to its potential as a tasty and environmentally-friendly food source, products will need to contain both fat and muscle arranged in a meat-like 3D structure. For example, the “marbling” found in a steak is an important feature that influences its quality and acceptability. Reproducing this type of patterning is, therefore, essential for many types of cultivated meat. Importantly, adipogenic and myogenic cells may mutually affect one another’s capacity for differentiation when co-cultured, though the exact details of this crosstalk are not yet fully elucidated (Pallaoro et al. 2023). Therefore, there may be advantages to differentiating each cell population separately and then combining them in a final processing step (Kawecki et al. 2023). However, Cheng et al. (2023) demonstrated the potential for co-differentiation of a porcine preadipocyte line with murine or porcine satellite cells to produce a “marbled” cultivated meat prototype without such a final processing step. This suggests that fat-muscle co-culture may be a feasible strategy for cultivated meat production, though production strategies employed in the lab may differ significantly from scaled manufacturing. Ultimately, the most successful strategies to produce high-quality products at a reasonable cost remain to be determined.
Explore known adipogenic lines
The table below includes adipogenic cell lines that are likely to be useful in cultivated meat or seafood research. Some lines may be available through publicly-accessible repositories or by contacting the cell line owner directly. You can use the “Filter” button to narrow down the list based on species, cell type, availability, or other criteria.
Myosatellite cells
The resident stem cell populations in adult skeletal muscle tissue are referred to as myosatellite or satellite cells. Satellite cells lie alongside myofibers under the basal lamina of the muscle tissue where they remain quiescent until activated upon injury or stress. In mouse, there are roughly 550 satellite cells per 1 mg of muscle tissue (Bentzinger, Wang, and Rudnicki 2012), making them one of the most abundant tissue-specific stem cell populations in the body. Satellite cells can be obtained from a small muscle biopsy, under local anesthesia or from animals recently slaughtered, and purified in the lab based on an array of well-characterized cell surface markers (Liu et al. 2015; Messmer et al. 2023). However, maintaining their proliferative capacity in vitro outside of the resident muscle niche has been challenging and is an area of active research. As a tissue-specific stem cell, activated satellite cells readily give rise to myoblasts, which eventually lead to the formation of myocytes, multinucleated myotubes, and myofibers, each delineated by the expression of key transcription factors (Chal and Pourquié 2017) and the activity of distinct signaling factors called myokines (Figure 4). There is some evidence that satellite cells can enter an alternative mesenchymal-like pathway, leading to the generation of other cell types such as adipocytes (Shefer, Wleklinski-Lee, and Yablonka-Reuveni 2004). Recently, it was demonstrated that satellite cells from Atlantic mackerel could take on an adipocyte-like phenotype in response to lipid supplementation (Saad et al. 2023). Somewhat speculatively, this may highlight a physiologic difference between fish and mammals, as at least in mice, the hypothesis that satellite cells can spontaneously enter into non-myogenic fates has been refuted (Starkey et al. 2011). Therefore, satellite cells offer the most direct method for obtaining skeletal muscle tissue in vitro, but it is unclear whether they might serve as a starting cell type for the creation of other cellular components of meat (Shaikh et al. 2021).
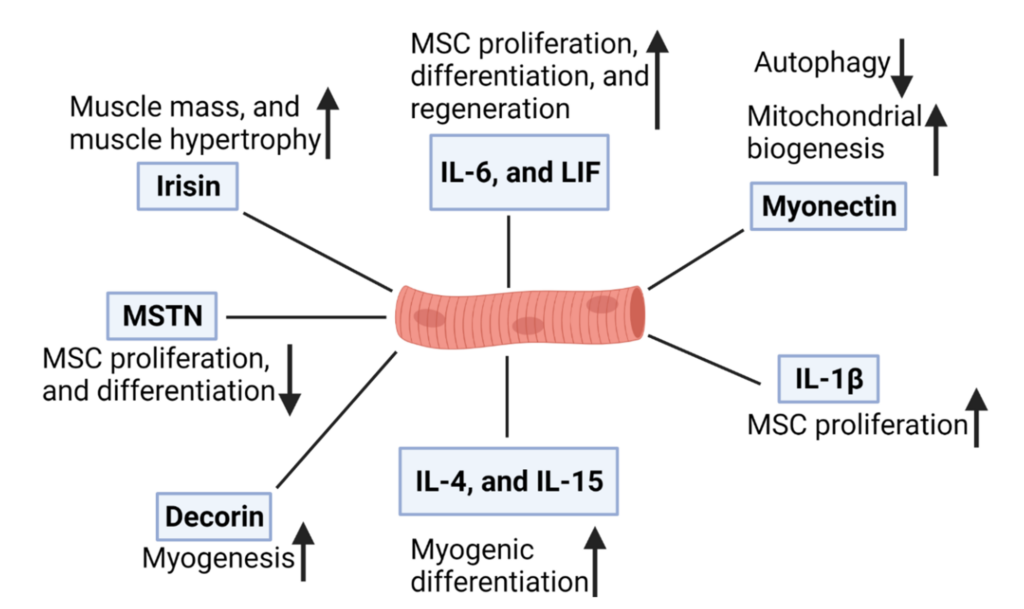
Figure 4. Some of the molecular signals that control the proliferation and differentiation of satellite cells. Reproduced from (Shaikh et al. 2021) under the terms of the CC BY license. Note that “MSC” is used here to mean “muscle stem cell.”
Methods for isolating and culturing satellite cells for use in cultivated meat have been reviewed by Choi et al. (2020). A common method for separating satellite cells from other cell types found in muscle is the use of a pre-plating step, in which a mixed cell population is plated, allowed to adhere briefly, and then the non-adherent cells are removed and plated again (Li et al. 2022; Ju et al. 2023; Čačković 2023; Kim et al. 2022). Because satellite cells are slower to adhere than other types, such as fibroblasts, this initially non-adherent population will be enriched in satellite cells.
Isolation and culture conditions for satellite cells
Recent studies on satellite cells from cow, pig, sheep, horse, chicken, turkey, and fish—many of them conducted with cultivated meat production as the intended application—have improved our understanding of the best isolation methods and culture conditions. Some of these studies are summarized below.
Cow
Main finding | Reference |
---|---|
Inhibition of the p38 pathway was successful in maintaining satellite cells in an undifferentiated, proliferative state. The effect was reversible, allowing for cells that had been maintained under these conditions to be differentiated into myotubes. | Ding et al. (2018) |
Isolation of CD29+CD56+CD31-CD45- cells by fluorescence-activated cell sorting (FACS) showed good results when initiating satellite cell cultures. Laminin-coated culture dishes showed the most promising results compared to collagen I and gelatin. Inhibition of p38 was not effective at maintaining Pax7 expression. | Čačković (2023) |
Several variables in the isolation procedure were compared, and the best results were found with mechanical disruption of the tissue following pronase treatment and plating on dishes coated with laminin 511. | Takahashi et al. (2022) |
The purity and viability of the satellite cell population could be improved compared to a previous isolation procedure published by the same research group (Lee et al. 2021). The variables optimized in the new procedure included the number and speed of the centrifugation step(s), the length of the pre-plating step, and the proliferation media used. | Yun et al. (2023) |
Storage of muscle tissue prior to cell isolation did not impair proliferation or differentiation capacity. | Skrivergaard et al. (2021) |
Satellite cells isolated from younger animals grew more quickly. | (Kim et al. 2023) |
Satellite cells from Holstein cows differentiated more efficiently than those of the Hanwoo or Jeju black breeds. | Kim et al. (2023) |
Satellite cells from bull calves proliferated faster than those isolated from dairy cows. | Skrivergaard et al. (2023) |
Expression of telomerase reverse transcriptase (TERT) and CDK4 was successful in immortalizing primary satellite cells. | Stout et al. (2023) |
Satellite cells were successfully grown on microcarriers in bench-scale stirred tank bioreactors. | Tzimorotas et al. (2023) |
Proliferation and differentiation capacity of primary satellite cells dropped over the course of the first ~ten passages. | Park et al. (2023) |
Pig
Main finding | Reference |
---|---|
More myogenic cells were isolated using the pre-plating method as compared to FACS. | Li et al. (2022) |
Myogenic cells could be isolated using either FACS for CD45-/CD31-/CD146+ cells or an explant-based model. | Dan-Jumbo (2022) |
Elevated temperatures reduced proliferation rates and seemed to transiently promote differentiation. The conditions tested included only constant temperatures (37, 39, or 41 °C), so it might be interesting for future studies to test the effects of a brief elevation in temperature at the start of the differentiation phase. | Park et al. (2023) |
Sheep and horse
Species | Main finding | Reference |
---|---|---|
Sheep | Myogenic and fibroblast cells were isolated using explants and enzymatic dissociation. | Andriessen (2021) |
Horse | Myogenic cells could be successfully isolated from adult horse muscle using an enzymatic dissociation protocol, though their proliferation capacity declined over time in culture. | Aimaletdinov et al. (2022) |
Chicken and turkey
Species | Main finding | Reference |
---|---|---|
Chicken | After comparing various combinations of temperature and time, a pre-plating method using 4 hours at 41 °C was shown to be most efficient for chicken satellite cells. | Kim et al. (2022) |
Chicken | In response to ammonia treatment, chicken myotubes express glutamine synthetase, show increased intracellular glutamine concentrations, and increase in diameter. The same phenomenon was not observed in mammalian myotubes. | Stern and Mozdziak (2019) |
Chicken | Although myogenic cells isolated from male and female chicken embryos showed some differences in expression of certain muscle markers, they were similar in their overall capacity for proliferation and differentiation. Because male embryos and fetuses are often discarded by the conventional meat industry, the authors suggested that male embryos might be a practical source of cells for cultivated meat. | Ju et al. (2023) |
Chicken | Chicken satellite cells cultured at 41°C showed better proliferation, differentiation, and higher umami flavor in the final product compared to those cultured at 37, 39, or 43°C. | Kim et al. (2023) |
Turkey | Turkey myogenic cells could be cultured for up to a year without loss of proliferative capacity, and were shown to express TERT. | Narenji et al. (2021) |
Fish (various species) and crustaceans
Species | Main finding | Reference |
---|---|---|
Croaker | Myogenic cells could be isolated from large yellow croaker using collagenase/trypsin treatment. Proliferative capacity was maintained for at least 26 passages, and cells could form myotubes. | Xu et al. (2023) |
Mackerel | A continuous cell line was established from Atlantic mackerel. Interestingly, the cells were capable of not only myogenic differentiation, but also of taking on an adipocyte-like phenotype when stimulated with lipids. | Saad et al. (2023) |
Goldfish | A continuous, spontaneously-immortalized myogenic line from goldfish was established using partial trypsinization combined with explant culture. | Li et al. (2021) |
Flounder | Satellite cells were isolated from olive flounder using either collagenase digestion or the explant method, the former of which produced the best results. Spontaneous immortalization was observed. | Krishnan et al. (2023) |
Carp | Cells were isolated from rohu carp muscle using the explant method, and were maintained for at least 38 passages. | Goswami et al. (2023) |
Catfish | Primary cells were isolated from walking catfish muscle using the explant method, and were maintained up to 13 passages at the time of publication. | Dhivyakumari et al. (2023) |
Rockfish | Myoblasts were isolated from rockfish using the explant muscle and cultured for at least 50 passages. | Kong et al. (2021) |
Lobster | Enzymatic dissociation using collagenase followed by trypsin was successful in isolating primary muscle cells from lobster. Satellite cells were shown to be more abundant in lobster larvae as compared to adults. Immortalized cells were not reported in this study. | Jang et al. (2022) |
Explore known myogenic lines
The table below includes myogenic cell lines that are likely to be useful in cultivated meat or seafood research. Some lines may be available through publicly-accessible repositories or by contacting the cell line owner directly. You can use the “Filter” button to narrow down the list based on species, cell type, availability, or other criteria.
Cell banking
A reproducible and consistent cell line is essential for any bioprocess. Due to the large number of cell population doublings needed to make cultivated meat at scale, there exists a sizable concern for genetic drift and cell line stability, which may lead to inconsistencies in downstream processing and final product. In essence, as cells continue to divide and replicate their DNA, the probability of an increased burden of genetic variation (single nucleotide polymorphisms, copy number variation, large insertions or deletions, epigenetic changes, or aneuploidy) also increases. In some cases, these variations can be harnessed for improved processing (e.g., adaptation to suspension culture or lower concentration of growth factors), however, in general, genetic stability is favorable for a reproducible process.
In order to mitigate the risk of genetic drift, cells will need to be initially expanded, validated via rigorous quality control, and cryopreserved as a master cell bank. Individual vials from the master bank can then be serially subcultured to produce working cell banks. Animal-origin free and chemically-defined cryopreservation techniques will need to be optimized for a diverse set of cell types from various species. This strategy can allow for batch processing of cultivated meat or continuous processing of progenitor stem cells, at least until sufficient differences are detected wherein the culture can then be restarted. Similar strategies are already employed in biomedical industries, such as vaccine production, and these may serve as a guide for the cultivated meat industry.
Thorough characterization of each cell line’s properties prior to banking will be advantageous, especially for lines with the potential to be directly commercialized in food products. Cell line properties can be broadly grouped into “ante factum” properties, which describe the origin of the cell line and “post factum” properties, which describe the observed characteristic of the line in vitro (Bols et al. 2023). For example, the species, breed, age, sex, and health status of the animal from which the cells were taken, the tissue of origin, and what methods and reagents were used during the isolation process would all be considered ante factum properties, while the doubling time, karyotype, cell type-specific gene expression, morphology, presence or absence of viral contamination, and response to culture conditions would be considered post factum properties. With adequate characterization, it is possible that cell lines previously banked for other purposes can be shown to be safe and reliable as starting cells for cultivated meat. For example, the chicken cell line used by GOOD Meat was originally banked in 1996.
Currently, there exist few publicly available cell lines of the aforementioned cell types from agriculturally-relevant species for cell-based meat production. Thus, there is a great need for the creation of new cell lines that can be banked and distributed to researchers in academia and industry alike. These biorepositories can be set up similarly to those dedicated to housing tissues and cell lines for efforts related to endangered animal conservation or large scale distribution networks (e.g., American Type Culture Collection). Having many cell lines available from a diverse array of animal species and breeds will be one of the most important factors in bootstrapping the research required for the cultivated meat industry to thrive long-term.
Sharing of cell lines between cultivated meat companies and academic researchers is gaining momentum along with an increasing number of B2B cell line providers emerging globally, as shown below. For example, the cultivated meat contract development and manufacturing organization (CDMO) Extracellular, in collaboration with the B2B media supplier Multus Biotechnology, launched a license-free cell bank in June of 2023, beginning with primary cells from cow, pig, and lamb. This and similar cell line sharing efforts may play a pivotal role in the success of the cultivated meat industry by reducing duplication of effort and allowing cultivated meat companies to focus their time and resources on improving the quality and scalability of their products
B2B cell line provider | Location |
---|---|
Opo Bio | New Zealand |
MyoWorks | India |
Neat Meatt Biotech | India |
ProFuse Technology | Israel |
Roslin Tech | UK |
PluriCells | UK |
Extracellular | UK |
Dragon Bio | UK |
Quest Meat | UK |
Livestock Labs | US |
Fisheroo | Singapore |
The Good Food Institute has partnered with reagent provider Kerafast to house cell lines relevant to cultivated meat in their existing biorepository. In a separate but related effort, GFI maintains a database of existing cell lines relevant to cultivated meat that can be ordered from various repositories or requested directly from the cell line owner. Please e-mail cells@gfi.org if you want to learn how to deposit your own cell line in the Kerafast repository, have a suggestion for an existing cell line that you’d like to see housed in the repository, or know of a cell line available from any other source that you think the cultivated meat research community should be made aware of via the database.
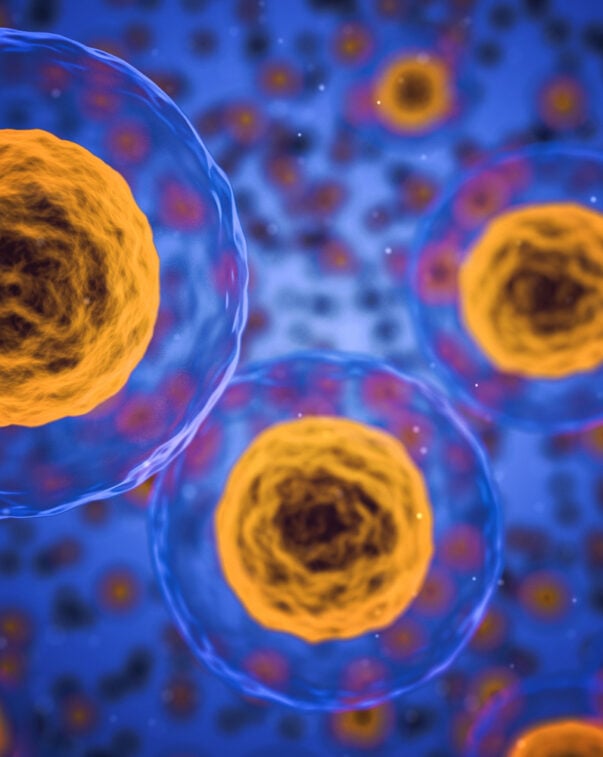
Resource
Expanding access to cell lines
Limited access to cell lines is a significant challenge for cultivated meat research. GFI is working to expand access and support the development of new cell lines.
Transdifferentiation
It is possible to directly convert (i.e. transdifferentiate) differentiated adult cell types such as fibroblasts into muscle (Ito et al. 2017), fat (Wu, Jin, and Gao 2017), or other cell types using similar techniques to those used to reprogram such cells into iPSCs. This approach is used by Israeli cultivated meat company Believer Meats, which uses fibroblasts as their starting cell type. A recent study published by scientists at the company described their method for inducing adipogenesis in spontaneously immortalized chicken fibroblasts (Pasitka et al. 2022).
Transdifferentiation strategies are somewhat limited in that conversion can be variable and incomplete, and they result in a post-mitotic population that undergoes limited expansion (Prasad et al. 2017). Given that the production of cultivated meat will require excessively large numbers of cells, proliferation would have to occur prior to transdifferentiation and all non-converted cells would be wasted, impacting the overall efficiency of the bioprocess. A viable transdifferentiation-based strategy would therefore rely on either 1) a highly efficient transdifferentiation process, or 2) a method for separating out transdifferentiated cells for downstream use while allowing the remaining cells to undergo additional cycles of proliferation and attempted transdifferentiation (assuming that efficiency does not decline in these subsequent cycles).
Jeong et al. (2022) demonstrated that two populations of bovine fibroblasts could be simultaneously transdifferentiated under the same conditions into myotubes and adipocytes. In this case, myogenic differentiation was achieved using an inducible TetOn system to express MyoD in the presence of doxycycline, while adipogenesis was induced by exposing PPAR𝛾2-expressing cells to oleic acid. The ability to trigger both myogenesis and adipogenesis from a mixed population of starting cells in the same medium would be advantageous for cultivated meat production, especially for whole cut products.
For cultivated meat applications, it may be advantageous to pursue reprogramming technologies using non-integrating or transgene-free methods to avoid potential regulatory issues with transgenes. For example, Qabrati et al. (2023) reprogrammed mouse fibroblasts to a myogenic fate by overexpressing MyoD via an mRNA construct, together with treatment by compounds that suppress key signaling pathways.
While transdifferentiation is often understood to refer to methods where over-expression of one or more transcription factors or other genes is directly induced as a means of triggering a change in cell fate, transdifferentiation-like changes without any direct genetic manipulation have also been described in some species. For example, chicken fibroblasts can be efficiently (>80%) transdifferentiated to an adipogenic-like fate by the addition of soy lecithin to the culture media (Pasitka et al. 2022). Chemical methods for transdifferentiation have also been described in mice, which could provide a starting point for the development of similar methods for food-relevant mammalian species (Qabrati et al. 2023). Similarly, a muscle cell line from Atlantic mackerel was able to take on an adipocyte-like phenotype capable of lipid uptake in response to lipid supplementation of the culture media (Saad et al. 2023). Additionally, a fibroblast-like line from filefish was able to take on phenotypes resembling skeletal muscle cells, adipocytes, and neural cells in response to changes in culture conditions, though it is important to note that the precise identity of these altered cells was not conclusively shown through molecular methods (Tsuruwaka and Shimada 2022; Tsuruwaka et al. 2015). If transdifferentiation—and especially transdifferentiation triggered by food-safe chemical methods alone—does turn out to be a viable method for cultivated meat production, this would be an especially helpful development in the case of fish and seafood. In contrast to the small number of terrestrial species commonly eaten by humans, a wide variety of species are represented in modern seafood production. While myogenic, adipogenic, or pluripotent cell lines exist for very few of these species, fibroblast-like lines exist for many species of fish (Thangaraj et al. 2021; Kumar et al. 2023).
Species may differ in their susceptibility to transdifferentiation, or in the exact protocols that will be most effective. In contrast to human and mouse fibroblasts, which can generally be transdifferentiated to muscle simply by expression of MYOD1, pig fibroblasts required a combination of MYOD1 overexpression and a cocktail of signaling molecules (Jeong et al. 2021). Even in mice, myogenic reprogramming of fibroblasts is not complete based on an analysis of gene expression, and the chromatin regulator CTCF was identified as a key mediator of the stable chromatin structures responsible for maintaining these cells in this intermediate state (Ren et al. 2022). Encouragingly, transient knockdown of CTCF at the same time as MyoD overexpression was shown to be effective in promoting a more complete transdifferentiation process, and the same method was effective in pig and chicken fibroblasts.
It is important to note, however, that what degree of reprogramming is considered “acceptable” may differ depending on the intended application. In the case of cultivated meat, it is essential that methods used for transdifferentiation are effective at producing a final product with the expected nutritional and organoleptic properties. Understanding the gene expression signatures that correlate with such outcomes is helpful on an experimental level, but ultimately it is likely that only a subset of the differentially expressed genes will be essential to the acceptability of the transdifferentiated muscle fibers and adipocytes as a food product.
Species differences
Much of the knowledge for culturing stem cell lines has come from the fields of cell-based therapy and regenerative medicine. While there are many fundamental similarities in laboratory techniques, protocols, and reagents that can be used in cultivated meat production, an important difference lies in growing cells from different animal species. The vast majority of published literature using the cell types previously described comes from studies in human and mouse. While there exist examples of studies using the stem cells described from bovine, porcine, ovine, avian, and piscine animals, the field as a whole lacks well-established protocols and the degree of rich scientific literature from which to draw upon. This is especially true in the case of fish and shellfish (Rubio et al. 2019; Bomkamp et al. 2023; Yoshino et al. 2013).
Despite this, a vast amount of information, mostly held privately by corporations, does exist on the cellular biology and genetics of livestock species, which may be leveraged for adapting livestock species for cell culture. Additionally, resources such as sophisticated genome annotations, validated antibodies, and other -omics datasets will need to be generated. Lastly, while key developmental processes are generally conserved by evolution, it remains unclear the extent to which species differences will affect the success of applying human- or mouse-based cell culture strategies to evolutionarily distant species such as crustaceans or fish. For example, it has been demonstrated that mammalian, avian, and fish myogenic cultures show substantial differences in their metabolic responses to the presence of ammonia in the culture media (Stern and Mozdziak 2019; Miramontes et al. 2020). As mentioned above, cells from different species have also been shown to differ in their susceptibility to transdifferentiation, requiring additional optimization for protocols developed in one species to be ported to another (Jeong et al. 2021). Thus, the overall bioprocess will likely be replicated from species to species, but key differences, either advantageous or disadvantageous, are to be expected due to inherent biological differences across an evolutionarily diverse set of species.
Cell line considerations
Intra-species variation, pathogen-free sources, and biopsy location
Other cell line selection strategies may entail generation of multiple cell lines from an individual animal, different biopsy locations within an animal, the age (Kim et al. 2023) or sex (Skrivergaard et al. 2023; Ju et al. 2023) of the animal, different individuals within the same species Kim et al. (2023) or breed, or clones of each derived cell line. For example, selective breeding has produced desirable traits in specific animal breeds and thus these same traits may be strategically recapitulated in vitro (e.g. using cattle from the Belgian Blue versus Holstein breed for skeletal muscle production) (Melzener et al. 2022). This has begun to occur in the cultivated meat industry, exemplified by US-based GOOD Meat (formerly JUST), which has partnered with the Toriyama farm in Japan to reproduce their renowned Wagyu beef. When possible, cell lines should also be obtained from animals that are raised in closed flocks, herds, or colonies, and free of known specific pathogens to reduce the risk of adventitious agents being present (Ju et al. 2023).
The location of an initial cell biopsy may also have downstream effects. As previously discussed, this could influence the differentiation potential of a mesenchymal stem cell population. For myosatellite cells, a muscle biopsy from a region of fast-twitch muscle fiber will in turn bias production of fast-twitch muscle fibers, which may influence final taste, texture, and metabolic rates of the cells in culture (Huang, Dennis, and Baar 2006). This may be especially true in fish, which typically show a more distinct spatial separation between fast and slow-twitch fibers. Generally speaking, fast-twitch or white muscle from fish is likely to be the main priority for cultivated meat applications, though there may be occasional exceptions. Location variables are less likely to influence pluripotent stem cell-derived outcomes, but individual and clonal variation amongst pluripotent stem cell lines is a notoriously difficult problem to manage (Kyttälä et al. 2016). With these considerations in mind, it’s likely that many species, stem cell types, and cell line variables will be explored in order to determine the best cell lines for cultivated meat production (Martins et al. 2024).
One of the inherent advantages of using cell culture to produce meat rather than an animal is the range of real-time testing, data analysis, and parameter iterations that can be made from biopsy to product packaging. For example, machine learning techniques have been used to predict proliferation and differentiation performance based on morphological parameters measured from phase contrast images of cultured porcine satellite cells (Wang et al. 2023). Such approaches could be used in the future to screen early-passage cells from different lots, animals, or breeds to identify those with the best predicted properties for cultivated meat without having to test their performance over multiple passages. These advantages can accelerate the rate of innovation to reach success and create new products versus traditional animal agriculture.
Food safety
As with any food product, ensuring the safety of cultivated meat production processes is and will continue to be critical. While food safety of cultivated meat products has been discussed elsewhere, it is worth considering in detail the food safety considerations specifically related to cell procurement, cell line development, and cell banking. Food safety considerations related to cell immortalization are discussed separately below. It is worth noting that cell lines were specifically mentioned by the US Food and Drug Administration (FDA) in its notifications to both UPSIDE Foods and GOOD Meat: “Should new production procedures, cell lines, or substances employed during production be used that could be relevant to the safety of the food, we strongly recommend that [company] consult with FDA to ensure that food resulting from any modified production process is in compliance with all applicable laws and regulations.”
An assessment by the Food and Agriculture Organization of the United Nations (FAO) and World Health Organization (WHO) identified a list of potential hazards throughout the cultivated meat production process (FAO and WHO 2023, see table 5 on page 78). Hazards directly associated with cell isolation included pathogens present in the initial biopsy and carried over into the end product, prions, allergens, antimicrobials and other veterinary drugs used during the biopsy step, microbial toxins, and novel allergenic or toxic substances produced as a result of genetic modifications. For each of these categories, the assessment also identified potential control measures, which are broadly grouped into mitigation-based and testing-based measures. For example, for the potential hazard of pathogens from the donor animal causing contamination of the final product, possible mitigation measures include requiring health certification for the donor animal, performing a health inspection on the animal prior to the biopsy procedure, using antimicrobials during sampling, and keeping the sample cold to prevent the growth of pathogens. In addition to mitigation measures, testing measures are also recommended to confirm the absence of viruses, prions, and other pathogens prior to cell banking. Related to cell banking, the assessment also identifies the potential for reagents used for cryopreservation to cause safety issues, and similarly recommends mitigation and testing measures that can be used.
Documentation submitted by cultivated meat companies to FDA provides concrete examples of how such measures may be implemented, especially when it comes to testing. In the case of bacterial and viral contamination, the results of testing measures—such as PCR and immunoassays to detect mycoplasma, viruses, and other pathogens—are reported (Licari 2022; Schulze 2022).
Prions—misfolded proteins that can induce misfolding in other copies of the same protein—are one possible hazard, though again conventional meat shares this hazard and there are existing measures and regulations in place (FAO and WHO 2023). The recommended strategy for preventing the introduction of prions into the cultivated meat supply chain is to obtain cells from healthy animals, avoid obtaining cells from tissues that are more likely to harbor prions, such as the brain or central nervous system, and testing to verify the absence of prions prior to cell banking. Relative to other species, prions are most likely to be a risk in cultivated beef, since transmission of prions from cows to humans has been documented in the past, whereas prion diseases in sheep, goats, and deer have been observed, but do not appear to be transmissible to humans (Imran and Mahmood 2011). Prion diseases have not been seen in pigs, chickens, and fish, and so remain a purely hypothetical hazard for both cultivated and conventional meat from these species.
It has also been suggested that genetic drift in the cells used to cultivate meat could lead to changes in gene expression, leading to the presence of toxic or allergenic compounds (Ong et al. 2023). Fortunately, commonly-consumed animal species do not produce venom or other toxic compounds, and there is no evidence for the presence of “silent” metabolic pathways that would produce natural toxicants. This fact makes it possible to control this hazard by 1) avoiding sourcing cells from known toxin-producing tissues, in the case of species where this is a relevant concern (such as snake venom glands), and 2) testing for any known allergens or toxins in a given species. Ingestion further mitigates risk of venoms, which typically require injection into the bloodstream to have biological effects.
As the FAO and WHO’s assessment makes clear, most of the potential hazards that could be identified in the cultivated meat production process are not unique to cultivated meat, but rather are also potential hazards in other industries such as conventional meat, fermentation, and biopharmaceuticals. Fortunately, this means that developing robust Hazard Analysis and Critical Control Point (HACCP) practices for cultivated meat can borrow from existing frameworks and can largely rely on existing mitigation and testing strategies. A comprehensive FDA risk assessment of the safety of meat products derived from animals cloned using somatic cell nuclear transfer concluded that—at least for species for which sufficient data was available—meat and other products from cloned animals did not pose additional hazards beyond those associated with conventional meat (Rudenko et al. 2007). This assessment failed to find evidence of “hidden hazards” associated with meat from cloned animals, and suggested that the existing strategy of regular inspections to ensure that meat and animal products are derived from healthy animals should be followed. Similarly, cultivated meat manufacturers should ensure that cell lines are derived from healthy animals, as well as following relevant practices for hazard mitigation in their downstream processes (Ong et al. 2023; FAO and WHO 2023).
Finally, it is worth noting that cultivated meat and seafood may present some opportunities for food safety advantages related to cell line acquisition, quality control, and authentication. Mislabeling of species is a common problem in conventional seafood, with the potential harm to consumers ranging from labeling of low-value species as higher-value ones, to difficulty in ensuring that one’s food comes from sustainable and ethical sources, to health and safety risks. Species authentication in cultivated seafood is likely to be much more straightforward. The presence of environmental contaminants such as heavy metals or microplastics is also a concern in meat, and especially in seafood. As long as the culture media and other inputs used in the cultivation process are of sufficient purity, we can expect that such contaminants—if present in the starting cell population—would be diluted out over the course of multiple cell doublings, resulting in a final product with much-reduced levels of such contaminants.
Adherence to religious standards
For those who adhere to certain religious traditions, conventional meat can be eaten only if certain customs are followed in its production and preparation. A version of these same rules is likely to apply to cultivated meat as well. Muslims make up approximately one quarter of the global population. This means that the status of cultivated meat as halal or non-halal can be expected to impact its acceptability to a substantial fraction of people worldwide. While Jews make up a smaller share of the population (0.2% globally and 2% in the U.S.), there is substantial interest in kosher foods even among non-Jews, exemplified by the fact that 41% of U.S. packaged foods as of 2015 were certified kosher. For cultivated meat to be accepted by members of these religious communities and those who prefer foods with the associated certifications, it will be essential for cultivated meat producers to take these considerations into account when designing their production processes. This section will discuss the currently accepted but still evolving interpretation of the rules for halal and kosher meat as they apply to cultivated meat, as well as areas where additional guidance may be needed.
According to the American Halal Foundation, cultivated meat may be considered halal provided that its production adheres to certain standards: 1) the source cells cannot be derived from a non-halal species (e.g., cultivated beef might be halal, but cultivated pork will not be), 2) the cells must be derived from an animal slaughtered according to Islamic rites (e.g., cells isolated from a muscle biopsy of a living animal cannot be used in halal cultivated meat), and 3) the culture media and other inputs must be halal. The guidelines published in early 2024 by the Fatwa Committee of the Majlis Ugama Islam Singapura (MUIS)—the entity responsible for halal certifications in Singapore—are similar. For cultivated meat derived from ESCs to be considered halal, the slaughter of the mother animal may be required (Izhar Ariff Mohd Kashim et al. 2023; Hamdan et al. 2018). However, it is unclear how this applies in the case of ESCs derived from in vitro fertilization. The requirement for slaughter does not apply to fish, so biopsy-derived fish cell lines are likely permissible as a cell source (Izhar Ariff Mohd Kashim et al. 2023).
Similarly, statements as of early 2024 by Jewish religious authorities indicate that cultivated meat may be kosher, but that its status depends on the details of the production process. Ashkenazi Chief Rabbi David Lau’s ruling that the cultivated beef produced by Aleph Farms should be considered kosher relied on the fact that the source cells were isolated from a fertilized egg. Cultivated meat whose source cells were derived from muscle tissue—whether from a biopsy or a slaughtered animal—would have to be evaluated separately. While starter cells derived from the muscle tissue of animals slaughtered according to kosher practices are thought to be acceptable, it is not clear how other cell sources will be interpreted under Jewish law (Kenigsberg and Zivotofsky 2020). Similarly to the considerations regarding halal cultivated meat, cultivated pork products are unlikely to be considered kosher, considering that the Orthodox Union declined to endorse Impossible Pork—an entirely plant-based product—as kosher, simply due to its resemblance to and self-description as “pork.” As fish is not considered meat under Jewish law, cell lines from fish may not be subject to the same requirements as kosher terrestrial meat. The influence of serum and other culture medium additives on kosher status is not entirely clear. It has been suggested that this hinges on the question of whether media ingredients are interpreted as being “absorbed” by the cultivated cells or whether it is considered the “food” of the cells in a manner analogous to the food eaten by an animal (Kenigsberg and Zivotofsky 2020).
It is worth noting that there is precedent for the development of halal- and kosher-certified culture media for use in other industries. We might expect the same to happen for the culture media that will be routinely used in meat cultivation. In this scenario, questions about the permissibility of non-certified ingredients under various circumstances might become mainly academic.
Survey data indicate that compliance with kosher and halal certification standards is at least a consideration for most cultivated meat companies, though some companies place a higher priority on this than others (Figure 5). In both cases, >50% of respondents indicated that compatibility with certification standards was “preferred.” However, the same survey indicated that only a minority of respondents (18% for halal and 13% for kosher) confidently understood these requirements, and that many companies planned to use biopsies from live animals for at least some of their cell line development efforts.
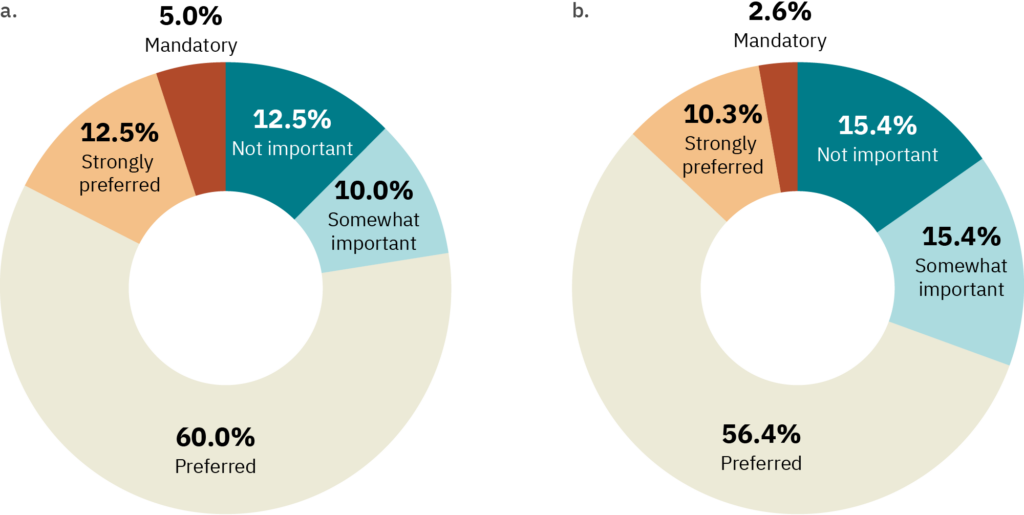
Figure 5. Results from a survey of cultivated meat producers. The survey questions were (A) “My company would rank cell lines that could comply with halal certification for relevant species as ______” n = 40 respondents, and (B) “My company would rank cell lines that could comply with kosher certification for relevant species as ______” n = 39 respondents.
In the absence of comprehensive guidance from religious authorities covering every possible variation on the cultivated meat production process, and in light of the fact that different religious sects and certification organizations may ultimately land on different interpretations, companies may choose to follow different strategies depending on the priority placed on kosher or halal certification. The Table below summarizes some of the cell line-relevant considerations for halal and kosher certifications for cultivated meat.
Halal | Kosher | |
---|---|---|
Cell source (ESC) | For in vivo fertilization, slaughter of the mother animal is likely to be required. It is unclear how this applies to in vitro fertilization. | Isolation from an in vivo fertilized egg may be acceptable (precedent from Aleph Farms), though there is still debate among religious scholars. There is no indication that in vitro fertilized eggs would pose additional challenges, and the fact that the fertilized egg is never inside the mother animal could make certification easier. |
Cell source (adult stem cell) | Cells should be sourced from animals slaughtered according to the appropriate religious rites. | Sourcing cells from animals slaughtered according to the appropriate religious rites may be the “safest bet,” but it is unclear whether this will be an absolute requirement. |
Species | Species for which the consumption of conventional meat is disallowed (e.g., pork) will similarly be disallowed in the case of cultivated meat. The requirements above for cell line sourcing are likely not to apply to species (e.g., fish) where religious rites are not required for slaughter. | |
Reagents used during cell line development | While the academic literature has included some debate regarding the permissibility of using serum during the establishment of a cell line (Hamdan et al. 2018; Kenigsberg and Zivotofsky 2020), avoiding the use of serum entirely during both cell line development and cultivated meat production is—at the very least—likely to be preferred. |
Cell proliferation and immortalization
In general, the process of cultivated meat production following cell line selection can be broken up into two phases: proliferation and differentiation. In the proliferation phase, stem cells divide repeatedly to generate a large number of cells until they are transferred to a new environment and triggered to differentiate into a mature cell type via changes in scaffolding, medium composition, or other aspects of the culture environment.
One hurdle in obtaining a large number of cells is that the number of times a cell can divide is inherently limited based on the Hayflick Limit. The Hayflick Limit imposes a limitation on cell divisions due to degradation of end-capping chromosomal telomeres following each cell division. Once a certain number of cell divisions occurs (typically around 30-50 in vitro for human cells), the cells enter a state of senescence and stop dividing. Thus, the number of potential cells acquired from a single starting batch is biologically limited. Some cells, however, can bypass the Hayflick Limit and achieve cell immortality. Identifying optimal strategies for producing immortalized cell lines with stable characteristics and verifying their food safety is a key priority for the cultivated meat industry (Soice and Johnston 2021). Pluripotent stem cells achieve immortality in part by epigenetic changes (Hochedlinger and Jaenisch 2015) and up-regulation of the enzyme telomerase (Huang et al. 2014), which prevents telomere degradation. This property makes pluripotent stem cells especially useful in initial scaling, although genetic drift during proliferation may also lead to cell senescence or apoptosis.
While some adult stem cells can retain some telomerase expression (Hiyama and Hiyama 2007), it is insufficient to acquire immortality. One alternative method is to rely on the accumulation of a sufficient number of genetic changes in vitro to bypass normal cellular checkpoints and achieve spontaneous immortalization. This strategy has been demonstrated in species including Atlantic mackerel (Saad et al. 2023), turkey (Narenji et al. 2021), and chicken (Schaefer-Klein et al. 1998; Pasitka et al. 2022). Many cell lines used in research were derived from spontaneously immortalized lines. However, the burden of genetic variation can also alter the biology of the cells in unpredictable ways, potentially limiting their utility. Additionally, biological variation between species or cell type can contribute to the probability of spontaneous immortalization. For instance, the naked mole rat, which is highly resistant to cancerous cell transformation, is hypersensitive to contact inhibition (Seluanov et al. 2009) and resistant to iPSC reprogramming (Tan et al. 2017). Other species such as lobsters, fish, and turkeys, which retain high telomerase expression (Klapper et al. 1998a; Klapper et al. 1998b; Gomes, Shay, and Wright 2010; Narenji et al. 2021), or elephants, which have multiple copies of the p53 tumor suppressor (Sulak et al. 2016), may thus be easier and harder, respectively, to achieve immortalization, although in vivo telomerase expression levels do not necessarily correlate to those observed in vitro (Venkatesan and Price 1998). These and other unique animal properties, known or currently unknown, may be strategically harnessed for cultivated meat production.
Having multiple cell lines will enable companies to choose lines that have the best downstream characteristics (e.g., proliferation rate, differentiation potential, etc), potentially avoiding labor-intensive strategies such as directed evolution to acquire the same characteristics. For example, cellular proliferation can be boosted by naturally-occurring variation over time when selective advantage is conferred from variants associated with cell growth. By sequencing large batches of human pluripotent stem cells, growth advantages were observed to be conferred by chromosomal aberrations (International Stem Cell Initiative et al. 2011) and the acquisition of dominant negative mutations in p53 (Merkle et al. 2017). While it remains to be seen if these specific aberrations will naturally occur in non-human stem cells, it is nevertheless the case that selected stem cell lines from clonal populations or multiple individuals will likely harbor unique variation that drives their utility in the cultivated meat bioprocess.
A targeted immortalization approach can be achieved by over-expression of exogenously added genes or viral proteins. In these cases, the over-expression of telomerase (Schulze 2022) in combination with inhibition of cell cycle genes such as p16 or Rb (Tsutsui et al. 2002), over-expression of other cell cycle genes such as CDK4 (Stout et al. 2023), or use of viral elements such as the SV40 Large T Antigen (Jha et al. 1998; Biegler et al. 2022) or adenovirus type 5 E1 gene (Sieber and Dobner 2007) have been utilized for cell immortalization. Precision gene editing (e.g. CRISPR) methods can be used in combination to insert these genes into safe harbor loci (Sadelain, Papapetrou, and Bushman 2011), minimizing the risk of alterations in global gene expression. Additionally, tandem use of genetic engineering strategies can create ON-OFF switches for immortalization, through use of Cre-lox or Flp-FRT recombination (Robin et al. 2015; Westerman and Leboulch 1996) or piggyBac transposon (Xie et al. 2016) systems. Thus, immortalization can be readily targeted or reversed. However, it is currently unclear how spontaneously immortalized or intentionally immortalized lines will be regulated, as significant genetic alterations and use of genetic engineering may warrant exclusionary regulatory standards, especially in certain countries.
Thus far, it is unclear whether spontaneous or targeted immortalization will ultimately be the favored method for cultivated meat production. Of the two companies with cultivated meat products on the market as of late 2023, documentation submitted by UPSIDE Foods to the FDA indicates that spontaneous immortalization is their preferred method, while over-expression of telomerase (TERT) is used in some cases (Schulze 2022, see page 20). GOOD Meat, meanwhile, uses a commercially available, spontaneously immortalized line (Licari et al. 2022, see page 10). Survey data similarly indicate a preference for spontaneous immortalization (79% of the companies who responded indicated that they would use this method) compared to non-integrating expression (47%) or permanent genome alteration (29%) as the method to immortalize cell lines intended for commercial use (Figure 6). When asked about the use of immortalization methods for R&D purposes, companies’ responses were broadly similar (71% spontaneous, 47% non-integrating, 38% permanent genome alteration).
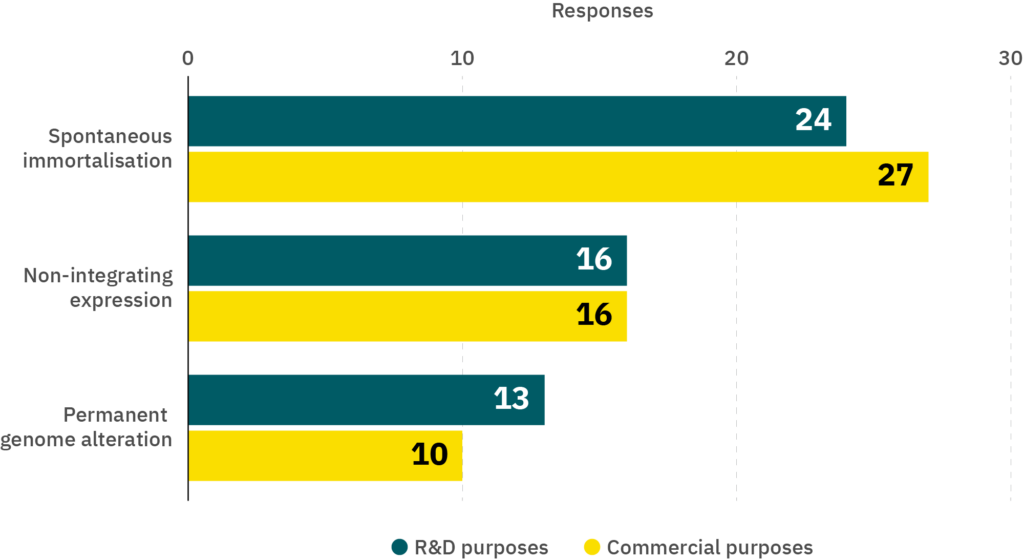
Figure 6. Results from a survey of cultivated meat producers. The survey question was “My company would use cell lines immortalised using the following methods (select all that apply).” n = 34 respondents.
Additional methods to improve proliferation rates or maintain proliferative capacity of non-immortalized stem cells exist. For example, in myoblasts, use of small molecule compounds can assist in maintaining proliferative capacity via targeting of proliferation pathways (Bar-Nur et al. 2018) or inhibition of specific proteins such as STAT3 (Tierney et al. 2014), p38 (Ding et al. 2018), and Setd7 (Judson et al. 2018). Other strategies include mimicking an injured or regenerative state via growth in the presence of cytokines (Fu et al. 2015), addition of small peptides normally released following exercise (Vinel et al. 2018), or growth of cells in a hypoxic environment, which may more closely mimic fetal oxygen levels (Liu et al. 2012). Similar strategies can tune biochemical pathways in other cell types such as adipocytes or chondrocytes. Many genetic engineering methods can also be employed to achieve similar results. These include gene over-expression via inducible or constitutively active systems and gene inhibition or knockout. In general, mimicking the native stem cell niche to retain stemness in vitro is an area of active research, and there exists a broad amount of possibilities for implementing these strategies for cultivated meat production.
Perhaps due to the fact that both processes involve rapid cell proliferation, as well as the overlap in the genes involved, concerns have been raised about whether consumption of immortalized cells used in cultivated meat production is equivalent to eating cancer and whether or not consuming immortalized cells is a food safety concern. First, it is important to note that modulating the balance between signals that promote and inhibit cell proliferation is a general mechanism that operates not only in the context of cancer but also in early development and wound healing. Furthermore, there is a fundamental difference between the chaotic, uncontrolled process of cell growth in the context of cancer and the rapid but tightly controlled process of proliferation followed by differentiation that would be desirable in the context of a cultivated meat production process. In other words, while the use of true “cancer cells” might be advantageous in the narrow sense that they would offer high proliferation rates and theoretically unlimited growth, this would come along with substantial downsides in the form of unstable cell phenotypes that would make designing a reliable bioprocess highly difficult.
Cancer cells can be described according to their acquisition of multiple hallmarks, of which insensitivity to the Hayflick limit is only one. Other hallmarks and enabling characteristics, such as loss of apoptosis, sustained angiogenesis, genome instability, metastasis, and evasion of the immune system, are likely to be either irrelevant or directly counter to the goals of cultivated meat producers. Thus, while all cancers are immortalized, not all immortalized cells are cancer, and there are fundamental differences between cancer cells and the type of highly-proliferative but phenotypically stable and signal-responsive cells that would be desirable for cultivated meat production.
Consistent with this, both the FAO (see section 4.4, page 110) and the U.S. FDA (see footnote 7, page 7) have expressed skepticism toward the idea that consumption of cultivated meat containing immortalized cells is likely to pose a food safety hazard. It is also worth noting that there have been no documented cases of cancer being transmitted through the ingestion of cancerous cells or tissues from non-human animals. Despite evidence suggesting that immortalized cell lines in general are safe, further research aimed at identifying and mitigating any possible food safety hazards associated with either immortalization procedures or the products of the immortalized cells themselves is still advisable (see section 3.2 of Soice and Johnston 2021).
Genetic engineering
Broadly speaking, genetic engineering refers to techniques used to manipulate the DNA of an organism. A variety of manipulations fall into this category, ranging from the insertion of genes from unrelated organisms (transgenics) to precise edits of single base pairs using the CRISPR-Cas9 system. It is important to note that genetic engineering is not a required part of the cultivated meat production process, but rather a tool that some companies or researchers may employ to achieve specific goals (discussed in more detail below). Genetic engineering strategies may be applied to cultivated meat for a variety of purposes. These include improvements to bioprocess efficiency, the removal of undesirable end product attributes found in conventional meat, or the introduction of positive attributes—or enhancement of existing attributes—to cultivated meat products.
Genetic engineering has already been applied in livestock species (Park 2023) and shrimp (Molcho et al. 2022), and early studies into its applicability to cultivated meat have shown promising results. However, the use of such techniques may come with additional regulatory hurdles. The exact details of the regulatory process will vary from region to region, and may differ depending on the exact technology being used (Mehta 2023).
Cultivated meat companies’ approach to genetic engineering spans the full range—from those who have committed to using only techniques that avoid the use of genetic engineering, to those who are exploring multiple avenues, to those who are proudly and enthusiastically embracing the opportunities afforded by engineering of cell lines. In a recent survey, 49% of the companies surveyed indicated that they do not use any genetic engineering methods at all, while only 21% indicated that they use genetic engineering for both R&D and commercial use (Figure 7). The remaining 31% use genetic engineering as a tool for R&D only.
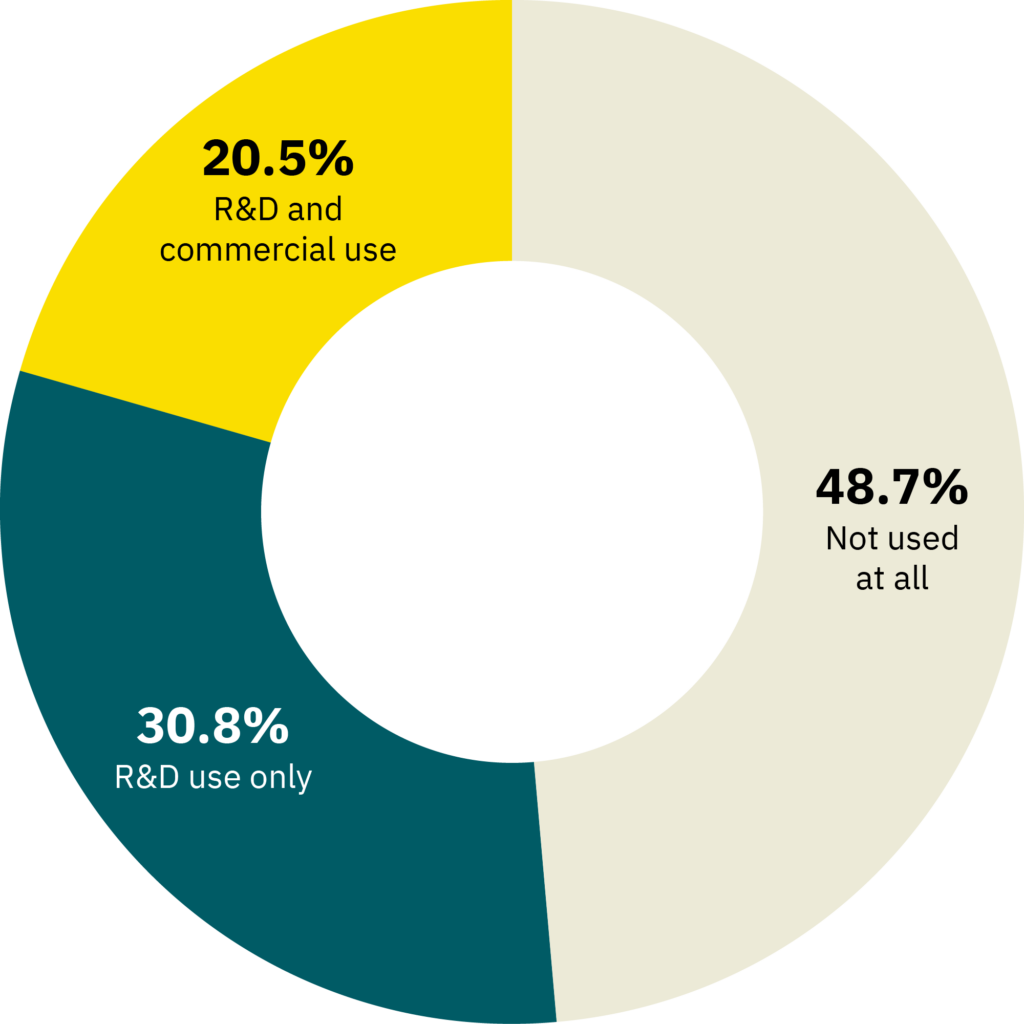
Figure 7. Results from a survey of cultivated meat producers. The survey question was “My company uses genetic engineering methods on our cell lines for _____.” n = 39 respondents.
Aleph Farms, GOOD Meat, and Vow have announced their intention to avoid the use of genetic engineering in their products—though Vow did use genetic engineering to create a “mammoth” meatball as a proof of concept that was not intended to be eaten. On the other end of the spectrum, both SciFi Foods and Meatable have touted the benefits of their cell line engineering approaches to develop lines that perform well in bioreactors and improve the speed of the differentiation process, and Atlantic Fish Co has stated that they are working to develop novel genetic engineering techniques. UPSIDE Foods received approval from the FDA for the overexpression of chicken telomerase reverse transcriptase as a strategy for cell immortalization. However, their filing also included two other immortalization strategies, and it remains to be seen which method(s) they will ultimately land on for their future commercial products intended for larger-scale production. The cultivated meat field collectively has a huge space to explore when considering both type of approach, and consumers may be more enthusiastic about genetic engineering approaches in some regions (e.g., the U.S., Japan, and possibly the UK) than others (e.g., the EU, Russia, India, Peru, and Venezuela, see page 12). Therefore, it is probably for the best that the field is not choosing to put all our eggs in one basket (cells in one bioreactor?).
Developing scalable bioprocesses for cultivated meat that will allow for products to be cost-competitive with conventional meat is undoubtedly a challenge (Humbird 2021). While some of these challenges will be best addressed by improved media formulations and bioreactor designs, others may be tackled by changing the properties of the starting cells themselves. For example, Stout et al. (2023) developed a bovine satellite cell line capable of growth in the absence of recombinant FGF2 (Figure 8). While the projected cost contribution of recombinant growth factors varies from study to study and depending on the modeled scenario, their contribution is often fairly substantial, especially when considering short-term scenarios (Humbird 2021; Vergeer et al. 2021). Therefore, reducing or eliminating the need for recombinant growth factors may offer part of the solution to bringing cultivated meat toward price parity with conventional meat. Similarly, cell lines with shorter doubling times, more tolerance for high cell densities or shear stress, or increased metabolic efficiency (Gomez Romero and Boyle 2023) might help lower the barrier to entry for cost-competitive cultivated meat. As mentioned above, engineering-based approaches are also one possible strategy for immortalization.
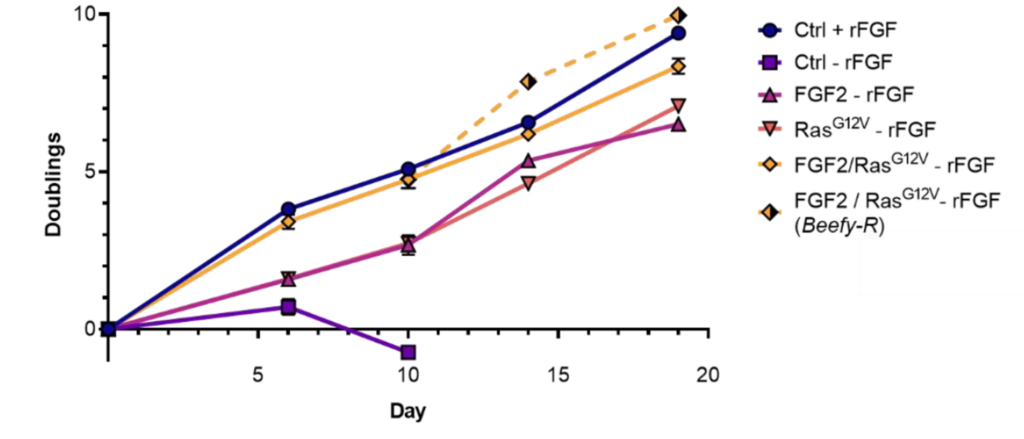
Figure 8. Example of the use of genetic engineering to improve bioprocess efficiency, in this case by removing the need for recombinant FGF2 in the culture media. Control cells did not grow in the absence of rFGF, while cells expressing FGF2, RasG12V, or FGF2/RasG12V continuously proliferated under the same conditions. FGF2/RasG12V cells offered equivalent growth to control cells with rFGF in both Beefy-9 and Beefy-R media. n=3. Reproduced from Stout et al. (2023) under the terms of the CC BY license.
Engineering approaches can also be applied to remove some of the negative health outcomes associated with meat consumption. While this is true of both conventional and cultivated meat, the technical challenge of doing so in cultivated meat may be somewhat easier because the modifications need to be made only in a cell line rather than in a whole animal (Park 2023). For example, allergies to a sugar called α-gal, which are often triggered by tick bites, can cause mild or severe allergic reactions to meat and other products derived from non-primate mammals. Removal of the GGTA1 gene (Choi et al. 2017), the product of which is responsible for α-gal production, from mammalian cell lines could open the door to cultivated meat that can safely be consumed by those with the α-gal allergy. Similarly, the presence of the sialic acid Neu5Gc in red meat has been linked to colorectal cancer (Samraj et al. 2015; Samraj et al. 2018). Removal of the CMAH gene, responsible for the production of Neu5Gc (Perota and Galli 2019), from cultivated meat cell lines could result in products that confer a lower risk of certain cancers.
Engineered cell lines could present opportunities to not only remove some of the “bad” things about meat, but to enhance the “good” things. Carotenoid synthesis genes have been introduced into bovine satellite cells, and the resulting lines showed both higher levels of the expected carotenoids and reduced oxidation during storage and cooking (Stout et al. 2020). Carotenoids are a class of antioxidants found in foods such as carrots and tomatoes, and their inclusion in cultivated meat products could introduce health benefits as well as improving the sensory characteristics of the product.
Additionally, while vertebrate animals including humans naturally lack the enzymes necessary for the synthesis of the nutritionally-important omega-3 fatty acids, introduction of two key desaturase genes fat1 and fat2 into zebrafish resulted in fish that could synthesize omega-3 fatty acids de novo and had substantially elevated concentrations of these fats in their muscle tissues (Pang et al. 2014). A similar strategy has also been demonstrated in mammalian cells (Zhu et al. 2014). Application of this strategy in cultivated meat or seafood could provide nutritional benefits and could also stave off potential future supply chain challenges. However, survey data indicate that such strategies have not yet been extensively explored by cultivated meat and seafood companies.
The combination of these two strategies (i.e., expression of the genes responsible for synthesizing both omega-3s and antioxidants) might also prove effective, as oxidation is a common challenge in omega-3-rich products. Such a strategy might offer “the best of both worlds” by producing products with the nutritional benefits associated with omega-3s without the downsides associated with their tendency to oxidize. Beyond these two simple examples, cell line engineering strategies could offer a wide variety of opportunities when it comes to optimizing the taste, texture, nutrition, and responses to cooking of cultivated meat.