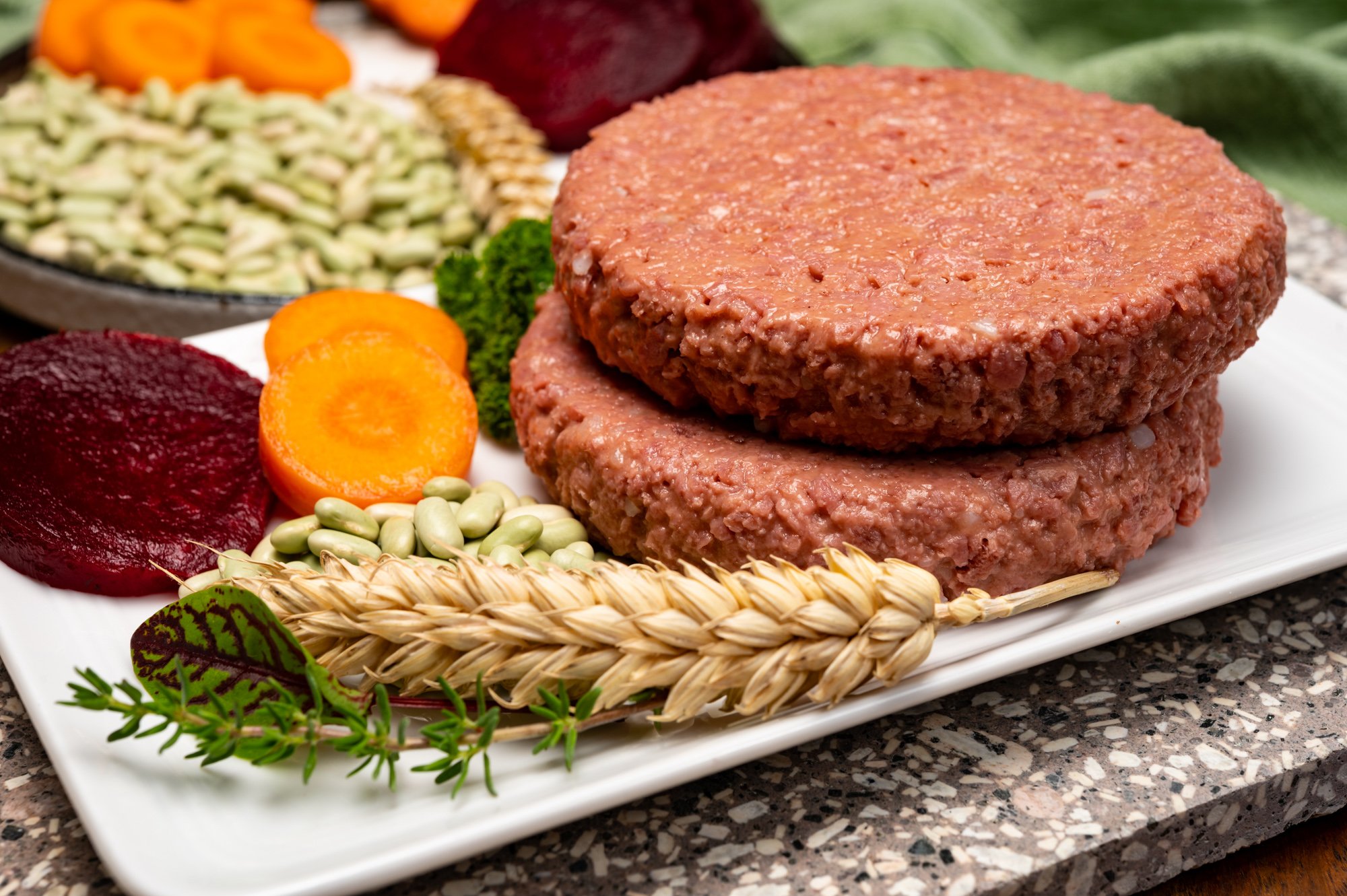
Deep dive: Plant-based meat end product formulation and manufacturing
Learn how plant-based ingredients interact when they are combined and about the manufacturing processes used to texturize plant-based meat.
End product formulation and manufacturing
Introduction
Plant-based meat producers strive to transform raw ingredients into products that replicate the cookability and organoleptic properties of restructured or whole-muscle conventional meat. Plant proteins are the main ingredients and display a wide range of functionalities. Other plant-based ingredients such as oils, carbohydrates, and nutrients bolster the end-product properties of proteins. Ingredient mixing and thermomechanical or biological manufacturing also significantly impact end product properties. This deep dive describes formulation ingredients, chemistry, and texturization. Figure 1 displays the typical process for plant-based meat products from textured vegetable protein hydration to packaging and storage.
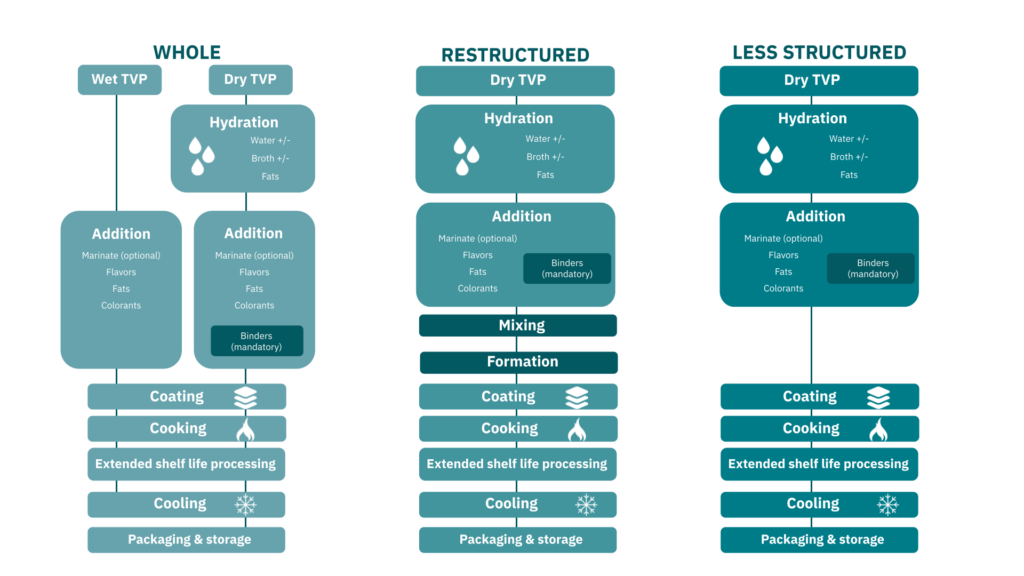
Source: GFI’s plant-based meat manufacturing guide.
Formulating with plant-based ingredients
Introduction to plant-based meat formulation
Plant-based meat aims to replicate or supersede not only the nutritional profile of animal-based meat but the whole consumer experience—organoleptic properties, stability, and familiarity in traditional meals. Formulating these products entails a sophisticated understanding of the complex interactions between ingredients. Inputs can be isolated raw ingredients—proteins, carbohydrates, oils, vitamins, and minerals—or complex materials such as flours, which are mixtures of proteins, carbohydrates, and more. Animal-free fermentation-derived or cultivated ingredients can enhance plant-based formulations. GFI’s Formulating with Animal-Free Ingredients provides an overview of ingredient processing and formulation development for plant-based, fermentation-derived, and cultivated products. GFI’s crop development and ingredient optimization deep dives explore how plant proteins are sourced and processed.
Components of plant-based meat formulations
Crops used in plant-based meat formulations are typically fractionated into enriched raw ingredients. Fractionation can mitigate batch-to-batch variation caused by field conditions, such as weather and soil quality, and improve ingredient functionality (e.g., removing antinutritional factors to enhance protein digestibility). Multiple opportunities to reduce the steps required to separate and recombine ingredients are available, such as breeding crops with optimized compositions and performing plant-based ingredient analysis and characterization. This section outlines the macromolecular components of crops used in plant-based meat formulations. The next section describes their functionalities and considerations for combining them.
Proteins
Plant proteins display a broad range of functionalities. They are typically “amphiphilic” (possessing both lipid-/fat-loving and water-loving properties) and globular, where the hydrophobic effect drives protein folding into 3D structures. In aqueous solutions, hydrophobic, non-polar amino acids (AAs) in the protein sequence are sequestered within the protein, while hydrophilic AAs interact with the external water molecules. Disruption of the protein structure results in non-polar and polar patches, which can impart emulsification, foaming, and gelation functionalities described below. Protein surface charge and exposed reactive groups also affect functionality. Relationships between protein sequence, structure, and functionality require further exploration. (See GFI’s solution on open-access databases with functional and characterization data.)
Optimized protein blends offer several formulation advantages, including more balanced essential-amino-acid compositions than single-source proteins. For example, wheat gluten has a low digestible indispensable amino acid score (DIAAS) of 48 and is limited in lysine, whereas pea protein has a DIAAS of 70 and is limited in methionine and cysteine (Herreman et al. 2020). Since their amino acid compositions complement each other, a 40:60 wheat-pea mixture has an improved DIAAS of 85. (See GFI’s crop-development deep dive for more information.)
Blends can also optimize overall protein functionality. Rice proteins can be combined with soy protein for enhanced water solubility, as well as emulsifying and foaming capabilities (Wang et al. 2013). A typical blend is soy protein and wheat gluten because of the high DIAAS and availability of soy protein and the superior gelation abilities of wheat gluten. In 2020, 14 of the top 25 plant-based meat products by U.S. dollar sales contained a soy-wheat blend. However, the scientific literature indicates plenty of room for composite optimization—soy protein isolates can negatively impact gluten gelation (Bainy et al. 2010), and gluten can lower the water-holding capacity of soy protein (Cornet et al. 2020). Evaluating novel protein composites will result in superior end product properties. GFI’s plant protein primer has information on the functional properties of proteins from 20 crop sources and briefly discusses synergistic combinations.
Fats
Fats include plant-based oils, the fatty tissue of animals, and any other compound or mixture of compounds that are esters of fatty acids. Because fatty acids are long chains of carbons, fats are strongly water-repelling, or “hydrophobic.” In conventional meat, fats improve juiciness, tenderness, and palatability. Because plant-based formulations tend to be made in aqueous conditions, incorporating fats has been challenging. An obstacle to making marbled meat has been creating solid fat differentiated from protein and with a melting temperature gradient above room temperature. Emulsification and oleogel formation, described below, can stabilize and modulate the melting temperature.
Higher in unsaturated fats, plant fats are generally healthier. Most animal fats contain high levels of saturated fats, which raise the risk of heart disease and stroke. An exception is unsaturated omega-3 fatty acids. Associated with improved health, omega-3s are highly concentrated in fatty seafood. Developing scalable, inexpensive animal-free omega-3 sources and incorporating them into alternative meat are active areas of research. Yield10 and Rothamsted are producing a CRISPR-edited camelina crop as a source of plant-based omega-3 fatty acids. Plant oils and omega-3s are unsaturated fats that are prone to oxidation, which negates their health benefits and creates off-flavors and unpleasant odors. Stabilization of these fats to prevent oxidation is a crucial need for alternative meat and seafood.
Structurally, animal and plant fats differ as much as animal and plant proteins. Animal adipose tissues are composed of fatty cells in a collagen fiber network, making their structure challenging to replicate with plant fats. Additionally, saturated fats are straight, single-bonded carbon chains. Unsaturated fats are kinked because their carbon chains contain double or triple bonds. As a result, saturated animal fats form more aligned, packed structures with higher melting points than unsaturated plant fats.
Animal fats typically have longer carbon chains and thus higher melting points. At room temperature, animal fats tend to be solid and melt gradually with applied heat. Plant-based oils are typically liquid or semisolid at ambient temperatures. More heavily saturated plant-based oils, such as cocoa butter, coconut oil, and palm oil, melt closer to ambient temperatures (~25ºC), so they may be partially solid in most climates. Plant-based fats that are solid at room temperature and exhibit a melting temperature gradient are extremely desirable.
Carbohydrates
Carbohydrates, another class of components found in plant sources, take the form of monosaccharides (single sugar units, e.g., glucose), disaccharides (two units), oligosaccharides (a few units), or polysaccharides (many units), the latter of which can be linear, branched, or coiled. Carbohydrates can be covalently bound to proteins, as described in the ingredient optimization deep dive, or added noncovalently as a bulking agent. Because carbohydrates are composed of many hydroxyl groups and some negatively charged groups (i.e., sulfur and carboxyl groups), they also bind to water. Carbohydrates can provide stability and impart a wide range of functionalities to proteins. Adding them to proteins shifts a formulation’s overall physicochemical traits, such as hydrophobicity, charge, and reactive groups.
Polysaccharides have interesting gelation and binding abilities because their hydroxyl groups interact readily with water and other compounds. Commonly used plant-based polysaccharides include starch, methylcellulose, xanthan gum, pectin, sodium or calcium alginate, carrageenan, and gum arabic. Table 2 in McClements & Grossmann 2021 breaks down common plant-based polysaccharides and their molecular characteristics. Studies of polysaccharide-protein interactions demonstrate a need for optimization. For example, iota carrageenan improved textural properties of soy protein, such as cutting force and elasticity, but decreased its cooking yield (i.e., the percentage of material mass after cooking as compared to raw material mass, which is related to water holding capacity) and expressible moisture (Palanisamy et al. 2018). Another study demonstrated that kappa carrageenan and konjac mannan improved the water holding capacity, texture, and cooking yield, while xanthan gum had no effect (Majzoobi et al. 2017). A recent review on applying starches to form fibrous meat analogs demonstrates the versatility of polysaccharides as a functional ingredient (Bühler et al. 2021).
Fibers
Native plant fibers are composed primarily of carbohydrates, usually with high concentrations of cellulose, while animal fibers are composed mainly of proteins (e.g., collagen and keratin). Dietary fibers are plant components that cannot be broken down by human digestive enzymes, including lignin, some polysaccharides, and some oligosaccharides. Plant fibers can enhance the viscosity, gelation abilities, stringiness, and nutritional profile of plant-based proteins. Depending on fiber solubility and concentration, adding plant fibers to proteins could impact downstream processes. GFI grantee Dr. Girish M. Ganjyal is exploring high-moisture extrusion of combinations of plant proteins and insoluble dietary fibers derived from legumes. Ganjyal’s lab previously explored extrusion of cellulose fibers (Kallu et al. 2017).
Mixing plant-based ingredients
Many parameters affect how ingredients interact with one another and their resulting functionalities. For example, ingredient solubility plays a significant role in formulation chemistry. Water-soluble ingredients are easiest to work with for mixtures because water is the preferred and safest solvent for food production. Protein solubility for many crops is discussed in GFI’s plant protein primer and crop development deep dive.
Phase-separated biopolymer mixtures can create anisotropic and fibrous structures in plant-based meat via shear force and heat stimuli (Figure 2, Dekkers et al. 2018; Dekkers et al. 2016). Incorporating fats is challenging because of their hydrophobic nature. Creating a stabilized, heterogeneous product, such as meat with fat marbling, is complex. Yet homogenously introducing plant fats to restructured meat products, such as sausages, patties, and nuggets, is also challenging. Mixed with water, fats and other lipophilic compounds separate from the water and other hydrophilic compounds. Phase-separated components can form a more homogenous system in an emulsion. Formulation component classes, emulsifiers, foaming agents, binders, gelling agents, flavoring agents, and coloring agents, and their functionalities are described in this section.
Emulsifiers and oleogels
Emulsions are mixtures of two (or more) immiscible liquids, with one liquid dispersed into the other. Foods such as salad dressings made from oil and vinaigrette are liquid-liquid systems that tend to separate over time and may require shaking to form a homogeneous dispersion of emulsions. Emulsifiers prevent such separation. They are typically amphiphilic molecules that effectively interact with oil and water phases. As a result, emulsifiers lower the surface tension between the oil-water interface, thus improving the kinetic stability of an emulsion. Because most proteins are amphiphilic, many have emulsification properties. For example, lentil, pea, and faba bean proteins were applied as emulsifiers to stabilize omega-3 oil (Gumus et al. 2017). Other plant-based emulsifiers include some polysaccharides (e.g., modified starch and gum arabic), phospholipids (e.g., soy or sunflower lecithin), and saponins (e.g., quillaja).
As mentioned, the homogenous and stable inclusion of plant fats into hydrophilic formulations is a challenge. Emulsion chemistry has been applied to overcome this challenge. To replicate animal adipose, sometimes oil-in-water emulsions are formed and crosslinked to stabilize the droplets. For example, canola oil was emulsified with soy protein isolate using high-shear dispersion. Then the protein-crosslinking enzyme transglutaminase was applied to form a plant-based emulsified fat crystal (Dreher et al. 2020a & 2020b). Emulsions can also encapsulate, stabilize, and deliver micronutrients (Zhu et al. 2021).
Oleogels are gels (semi-solid matrices) with high fat-holding capacity that provide a good alternative to solid fats (Co & Marangoni 2018). Vegetable oils benefit from emulsifiers in oleogel formation. Ethyl cellulose and hydroxypropyl methyl cellulose biopolymers have been applied to form oleogel shortenings of sunflower oil and palm stearin with high melting points (Naeli et al. 2021). GFI grantee Dr. Ricardo San Martin uses saponins to create plant-based oleogels. Dr. Martin’s lab developed a stable plant-based oleogel with a melting point of >82ºC (compared with 24ºC for coconut oil). Oleogels formed with proteins are especially appealing for plant-based meat (Feichtinger & Scholten 2020). There are many research opportunities to improve plant-based fat structuring and stability. (See GFI’s solution on fat and moisture encapsulation to stabilize formulations.)
Binders
Binders are compounds that hold together other components. This function can be achieved on a molecular level through several interactions, but typical mechanisms in food science include binding via cohesive or adhesive forces, such as hydrogen bonding or electrostatic interactions. Common binders used in plant-based meat include soy protein isolate, methylcellulose, carrageenan, and modified starches. Overall, these binders improve the thickening, gelation, and textural properties of end products.
Methylcellulose is an especially common binder. Polysaccharides generally have good binding abilities because the many hydroxyl groups engage in extensive hydrogen bonding. The nonpolar methyl groups are synthetically added to cellulose to generate methylcellulose, instilling distinctive gelation characteristics (Spelzini et al. 2005; Ismail et al. 2020). Methylcellulose forms firm gels when heated and melts into a viscous liquid when cooled, instilling a firm bite in cooked plant-based meat. This response differs from the thermo-responsiveness of many biopolymers, which often gel when cooled and liquefy upon heating. Because of these unique properties and good emulsification, methylcellulose is widely used with plant proteins to achieve desirable textures in cooked plant-based meat.
Recent years have seen an increased push to identify alternatives to methylcellulose that are familiar to consumers on ingredient labels. Fortunately, many proteins and polysaccharides act as binders. One study tested carrageenan, soy protein concentrate, casein, and xanthan gum as binders in mushroom-based sausage and found that carrageenan most improved textural properties (Arora et al. 2016). Crop sources contain soluble and insoluble fibers in varying amounts. Citrus fibers with enzymatically optimized pectin are a promising substitute for methylcellulose.
Gelling agents
Gelling agents promote formation of porous 3D networks with high water- or fat-holding capacities through non-covalent or covalent bonding. They act as binders and texturizers or encapsulate insoluble particles within their matrix (Cao & Mezzenga 2020). In the context of gelling hydrophilic biopolymers (i.e., proteins and polysaccharides), gelling agents typically increase water holding capacity by promoting disulfide formation, hydrophobic or ionic interactions, and other biopolymer interactions. Methylcellulose, other polysaccharides, and many proteins have good gelation behavior, especially with external stimuli that promote crosslinking, such as enzymatic treatment, heating, cooling, salting, or adjusting pH. For example, alkaline pH promotes wheat gluten gelation through disulfide and other AA bond formations (Li et al. 2018). Combining biopolymers with good gelation abilities can further enhance gelling properties. Oat protein possesses good gelation properties in alkaline pH at high temperatures (>110 ºC), but adding the gelling agent inulin, a non-digestible polysaccharide, improves gel strength at temperatures more suitable for efficient manufacturing (Nieto-Nieto et al. 2015).
Other common gelling agents for biopolymers include cationic mineral ions, which interact well with anionic protein AA groups and polysaccharides. Tofu salt coagulants have Ca2+ or Mg2+ divalent cations (e.g., calcium sulfate or trimagnesium citrate) that crosslink soy proteins with phytic acid (Joo et al. 2020). Monovalent ions, such as sodium ions, which improve heat- and cold-induced gelation abilities of soy protein isolate when added before spray drying (Zheng et al. 2019). This same study showed that divalent calcium and magnesium ions, Ca2+ or Mg2+, were more effective at relatively low concentrations.
A common biological gelling agent is transglutaminase, which catalyzes reactions between glutamine and lysine residues within and between proteins, thus enhancing the 3D structure. Transglutaminase has been used as a gelling agent for animal, soy, wheat, rice, pea, lupin, sunflower, and other proteins (Dube et al. 2006). (See GFI’s ingredient-optimization deep dive for more examples and further details about the crosslinking mechanism of transglutaminase.)
Flavoring agents
Flavoring agents impart desirable flavors or mask off-flavors to improve a formulation’s taste and odor. They are essential for plant proteins, which often have off-flavors and lack the aroma associated with conventional meat. For example, legume proteins have an unpleasant beany flavor due to lipoxygenase-initiated peroxidation of unsaturated fats (Shi et al. 2020). Off-flavors also arise through non-enzymatic browning Maillard reactions. Savory and meaty-flavored compounds, such as certain amino acids (e.g., cysteine, methionine, proline, and lysine), nucleotides, reducing sugars, and thiamin can improve flavor (Kyriakopoulou et al. 2019; Ismail et al. 2020).
A recent development is the use of synthetic biology to derive natural and novel flavor compounds that replace conventional synthetic and natural flavors. This technology helps produce flavors such as vanillin that are in high demand but require an unsustainable quantity of crops or petrochemicals to produce. This technology can produce flavor compounds for plant-based meats through fermentation. Note that flavor compounds can bind to plant proteins, significantly altering protein structure or lowering the flavor’s organoleptic effect (Moon et al. 2007a; Moon et al. 2007b). Additionally, proteins’ water- and fat-holding capacities impact flavor retention. Accordingly, optimization of the flavoring agent is necessary for various protein bases.
Flavoring agents can also sequester compounds that impart undesirable organoleptic properties. For example, cyclodextrin is a cyclic carbohydrate that can capture small hydrophobic molecules. Through this mechanism, cyclodextrin spray-dried with pea protein isolate mitigated the beany odor of the isolate without changing its structure (Cui et al. 2020). The need for flavoring and masking agents can be reduced by improving innate plant protein organoleptic properties through breeding or enrichment to select for lower levels of undesirable compounds.
Coloring agents
Coloring agents impart visual appeal to formulations and include plant pigments such as carotenoids, chlorophyllin, anthocyanins, and betanin. A common goal in plant-based meat formulation is mimicking the transition of pink or red to brown during heating. The mechanism in red meat, detailed in the crop development deep dive, occurs via heme proteins. Heat-unstable plant pigments, including red beet extracts, have replicated this effect in products such as the Beyond Burger. Reducing sugars have also been used so that the Maillard reaction occurs between sugars and proteins during cooking, resulting in brown color formation. Impossible Foods’ burger uses fermentation-derived coloring agents to produce its soy leghemoglobin.
These formulation components are relevant to plant-based meat as well as plant-based egg and dairy products, as discussed in GFI’s plant-based egg alternatives report and a recent review of plant-based milk by McClements et al. 2019. Other ingredients, such as thickeners, nutrients, antimicrobials, and antioxidants, are discussed further in other reviews (Sha et al. 2020; McClements & Grossmann 2021). Formulation components can be added before, during, or after texturizing proteins, depending on ingredient stability, manufacturing capabilities, and desired end product.
Texturizing and manufacturing plant-based meat
Introduction to plant-based meat texturizing and manufacturing
Animal skeletal muscle has a complex, hierarchical structure composed of fibrous protein bundles. Bomkamp et al. 2021 and Listrat et al. 2016 provide more detail on animal muscle tissue, and GFI’s crop development deep dive explores the functional differences between animal and plant proteins. Most plant proteins are globular, making the use of native plant proteins to replicate fibrous animal muscle structures challenging. Plant proteins must typically be denatured and unfolded before being aligned and crosslinked to create plant-based meat. This section describes several techniques for imparting texture to plant protein. See GFI’s 2020 state of the plant-based industry report for recent developments.
The desired texture of the end product depends on the type of conventional meat to be replicated. Restructured, including emulsion-based, products resemble shredded or ground meat products, while whole-muscle-cut products replicate striated muscle cuts from animals. Restructured products require more homogenous structures, whereas the fibrousness and juiciness of whole cuts owe to heterogeneous structures. Figure 2 illustrates the fibers produced by various texturization techniques and compares them to meat fibers and product sizes.
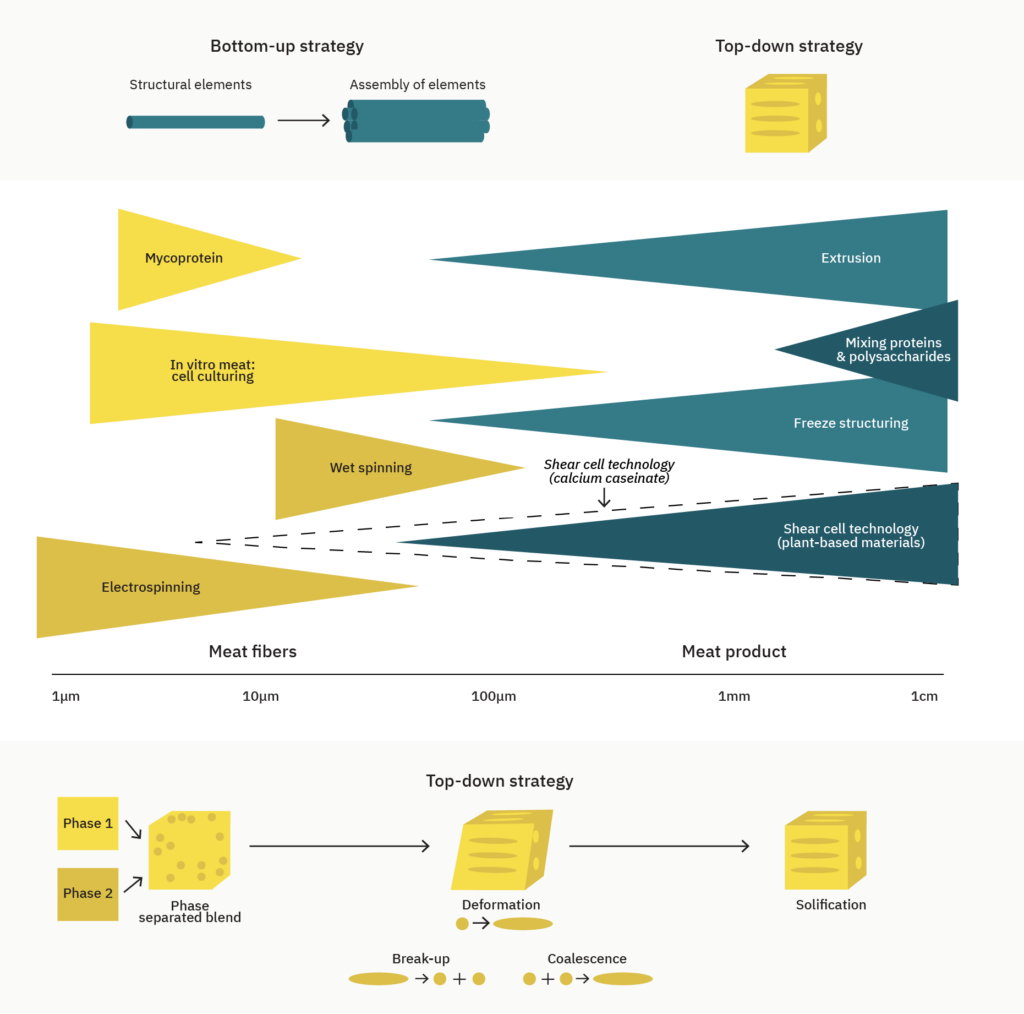
Source: Birgit L. Dekkers et al., “Structuring Processes for Meat Analogues,” Trends in Food Science & Technology 81 (November 2018): 25–36. Reprinted with permission from Elsevier.
Mechanical texturization methods
For a deeper understanding of the mechanisms that occur during biopolymer mixing and structuring, see Dekkers et al. 2018 and section 4.1 of McClements & Grossmann 2021. Cornet et al. 2021 goes into more detail about high-moisture extrusion and shear cell technology specifically.
Technique and co-ingredients determine the mechanism by which plant proteins texturize. When proteins and polysaccharides mix in water, the biopolymers are miscible, co-soluble, or separated into distinct phases. At the concentrations necessary to form plant-based meat, biopolymers generally separate into multiple water phases. With shearing and heating, multiphase mixtures can undergo water-in-water emulsification and further structural changes such that the dispersed phase is elongated in the direction of flow (Figure 2). The cooled resulting structure solidifies this matrix, forming fibrous structures.
“Top-down” methods of texturization, which structure biopolymer blends by applying external forces, will be discussed first here, followed by “bottom-up” strategies, which assemble fibrous structural ingredients to build an end product. Table 1 provides a summary of the methods.
Table 1. Texturization strategies to create plant-based meat analogues
Technique | Starting material | Commercial availability | Length scale anisotropy | Robust-ness | Scalability | Environ. impact |
---|---|---|---|---|---|---|
Top-down strategies | ||||||
Mixing proteins & hydrocolloids | Protein isolate, hydrocolloids, cations | Yes | ? | + | + | + |
Extrusion | Defatted protein flour, concentrate, or isolates blended with polysaccharides | Yes | Orientation on microscale | ± | ++ | ++ |
Shear cell | Protein concentrate or isolates blended with polysaccharides | No | ± | + | ++ | |
Freeze alignment | Protein isolate | No | + | ± | – | |
Bottom-up strategies | ||||||
Wet spinning | Protein isolate, coagulation bath | No | Micrometer | + | – | – |
Electrospinning | Protein isolate | No | Nanometer | + | – | – |
Low- and high-moisture extrusion
Extrusion is a mechanical process that transforms biopolymer formulations into continuous semisolids. An extruder consists of a motor that rotates a screw system within a barrel and forces inputs (e.g., dry ingredients such as proteins and starches, water, and oil) to mix thoroughly and then flow through a die (i.e., small opening), pressurizing and shaping the mixture as it exits (Figure 3). The screw system mixes the ingredients and applies shear forces and high temperatures that denature, unfold, and reorganize biopolymer structures. Twin screws that rotate in the same direction are preferred over single-screw systems because they have better mixing capability and, therefore, higher process productivity. Before entering the die, ingredients are typically melted using high temperatures (>100 ºC). Different types of extrusion apply varying amounts of heat, mechanical energy, pressure, and moisture.
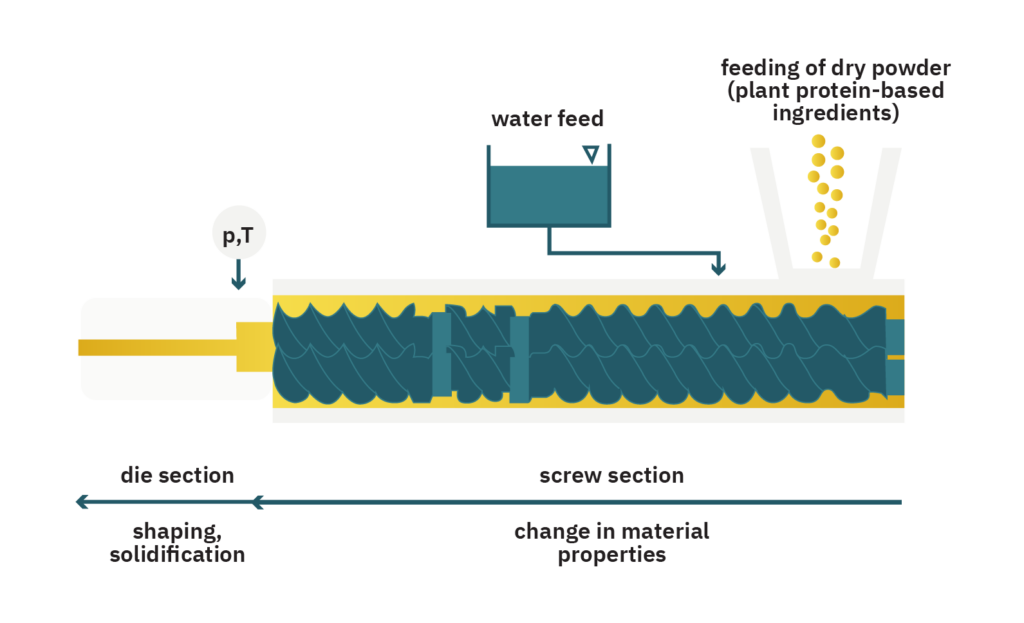
Source: Valerie Louise Pietsch et al., Combining Extrusion, Electron Microscopy and Rheology to Study the Product Characteristics of Meat Analog Products (Waltham, MA: Thermo Fisher Scientific, 2021). Used with permission from the publisher.
Extrusion systems are relatively well established. They have been scaled for high output of up to 500 kg/h (McClements & Grossmann 2021) and used with many plant proteins, although optimizing conditions for novel protein sources requires substantial troubleshooting. GFI grantee Dr. Zata Vickers is exploring twin-screw extrusion to form muscle-like structures with pulse proteins, which are increasingly desired by plant-based meat producers as alternatives to crops such as soy and wheat.
Low-moisture extrusion (LME, moisture <50% during texturization) and high-moisture extrusion (HME, moisture 50-70% during texturization) are the main types of extrusion used to texturize plant protein formulations. Dry textured vegetable protein (TVP) is generally produced through LME with dry ingredients and water, without added fats. It runs through a high-temperature die with a short nozzle (typically >100 ºC to evaporate water) and is further dried after extrusion to extend its shelf life. By contrast, high-moisture meat analog (HMMA) (sometimes referred to as “wet TVP”) is typically made with twin-screw HME with dry ingredients, water, and possibly fats. It runs through a long cooling slit die (20-80 ºC) that prevents water evaporation and thus retains high moisture. Low- and high-moisture extrusion can often be done with the same equipment, with interchangeable dies depending on the application. A breaker plate can be added before the die to enhance fiber formation (Cornet et al. 2021). Both LME and HME are highly dependent on process variables such as moisture content, screw speed, and die temperature and require extensive optimization.
Due to the quick temperature and pressure changes during LME, the food product tends to expand rapidly upon exiting the die. This process forms a puffy, crispy material with marked effects on protein structure and functionality. LME can remove antinutritional factors and has been shown to improve in vitro digestibility of lentil protein and starch (Rathod et al. 2016). Canola meal (Zhang et al. 2017), pea protein isolate (Beck et al. 2017), and dehulled black beans have also been subjected to LME (Alfaro-Diaz et al. 2021). In the third example, extrusion improved protein fractionation as a pretreatment.
HME uses more water and oil, enabling biopolymers to undergo deformation (Figure 2). As a result, HME forms more anisotropic structures than LME and is more often used to mimic conventional whole-muscle meat cuts. HME has been used at commercial scale for a number of soy- and wheat-based products, but academic investigations into HME with less well-characterized proteins inform adaptations of this process to new protein sources. For example, when peanut protein was processed using HME, it was found that the α-helical structuring dominated to form fibrous textures (Zhang et al. 2019). Additionally, the arachin protein fraction contributed to forming a fibrous structure more than the conarachin fraction. Subsequent studies demonstrated that many process parameters (e.g., moisture content, barrel temperature, shear strength, die pressure, die temperature, and other specific mechanical properties) affected peanut protein’s physicochemical and functional properties (e.g., color, fibrous structure, tensile properties, hardness, and springiness (Zhang et al. 2020a)). For example, temperature significantly altered tensile properties and springiness, while moisture content played an important role in color and hardness. The same research group also studied HME of peanut protein mixtures with carrageenan, sodium alginate, or wheat starch mixture (Zhang et al. 2020b). The polysaccharides imparted thermostability to the arachin and conarachin protein fractions and modulated end product strength, springiness, and fibrousness. Other examples of HME plant proteins that have been studied in the scientific literature include lupin (Palanisamy et al. 2018), soy protein (Pietsch et al. 2019a), wheat gluten (Pietsch et al. 2019c & Pietsch et al. 2019b), and soy protein-wheat gluten blends (Guo et al. 2020).
A comparison of wheat gluten processed by LME and HME found that HME-formed products had better stability of springiness and cutting strength (Samard et al. 2019). Extrusion is currently the most scalable texturization technique because it is a continuous process and has historically been applied to many other foods and nonfoods. Extrusion leaves ample room for optimization to enhance protein-fiber formation and reduce energy use. To better evaluate protein modification during extrusion, GFI grantee Dr. Filiz Koksel develops in-line sensors for plant-based meat extrusion. Meanwhile, GFI grantees Dr. David Julian McClements and Dr. Birgit Dekkers are studying texturization of plant proteins through nonextrusion methods, including shear cell technology. Extruded HMMA product is shown in Figure 4 and demonstrates an anisotropic, fibrous structure.
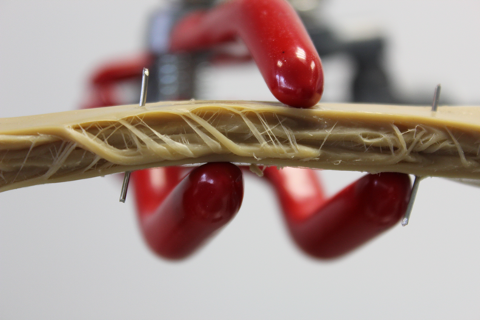
Source: Used with permission from Thermo Fisher Scientific.
Shear cell technology
Shear cell technology was initially developed as an analytical tool to model the effects of extrusion in a simplified system. Extrusion requires intensive mixing in the barrel, causing complex flow patterns and making the process challenging to analyze. The shear cell was developed as a rheological tool that could measure the effects of shear and heat on dense materials, such as biopolymers (van den Einde et al. 2004; Krintiras et al. 2014). Researchers then discovered that with the addition of crosslinking agents (e.g., transglutaminase and calcium) the technology itself could be used to texturize protein (Manski, van der Goot, & Boom 2007). As Dr. Birgit Dekkers, co-founder of Rival Foods, explains in an informative seminar, shear cell technology allows for independently controlled shear rate, temperature, and residence time, whereas extrusion couples these parameters. When Dr. Dekkers was in her graduate studies, she joined the team that developed shear cell technology at Wageningen University & Research with advisor Dr. Atze Jan van der Goot. The van der Goot lab has developed methods for plant protein texturization using shear cell technology, including for soy (Grabowska et al. 2016; Dekkers et al. 2016), pea-gluten and soy-gluten blends (Schreuders et al. 2019), and rapeseed (Jia et al. 2021) proteins. This work has been developed at pilot scale (Krintiras et al. 2016) and commercialized through the Plant Meat Matters consortium at Wageningen University & Research, with collaboration from industry partners including Avril, Ingredion, Givaudan, The Vegetarian Butcher, and Unilever. Rival Foods is the only company applying shear cell technology for commercial use. In collaboration with LIVEKINDLY Collective, Rival Foods is creating a plant-based chicken product through shear cell technology.
Shear cell machinery is designed in two primary configurations. The first uses conical shapes, where the bottom cone rotates and the top remains stationary, achieving a sealed gap and applying shear force on the middle protein layer. The second uses cylindrically shaped Couette cells, where the inner cylinder rotates and the outer remains stationary (Krintiras et al. 2016). Anisotropic structures of layered fat and plant protein form with the addition of a fat phase, demonstrating a mechanism similar to the one in Figure 2. Fibrous structures form most easily when ingredients with similar viscosities are used.
Shear cell technology also allows for increased material thickness (Dekkers et al. 2018). As a result, it is a promising technology for creating plant-based meat that more closely resembles whole-muscle cuts. Currently, shear cell texturization presents challenges in scalability and throughput because it is a batch process that requires premixing.
3D printing
3D printing, sometimes termed “microextrusion,” is another promising technology for forming whole-muscle structures from plant proteins. Companies such as Revo Foods, Redefine Meat, and NovaMeat use 3D printing, which can improve manufacturing precision and flexibility. Soy protein isolates mixed with sodium alginate and gelatin produced 3D-printed protein with improved hardness and chewiness (Chen et al. 2019). The 3D printing ability of soy protein isolate with xanthan gum (Phuhongsung et al. 2020)—where the addition of sodium chloride improved soy protein isolate printability—and pea protein hydrolysate with xylose (Zhou et al. 2020) have also been explored. While 3D printing is a continuous process that can form nano-sized, anisotropic fibers in flexible structures, it has been challenging to commercialize for plant protein texturization because of scalability limitations.
Freeze alignment
Also referred to as “freeze structuring,” freeze alignment can form anisotropic structures: Heat removed unidirectionally causes alignment of ice crystal formation. Quorn uses this approach for mycoprotein-based meat. Freeze alignment has been sparsely studied for plant proteins but could be a gentler alternative to the mechanical methods outlined above and require less capital-intensive equipment, thus lending itself to more rapid scaling. Further research is needed in this area.
Spinning
Various spinning technologies are being applied in the plant-based meat industry, including by Cybercolloids (high-shear spinning) and The Netherlands Organisation for Applied Scientific Research (electrospinning). Wet spinning for meat analogues has been patented since the 1950s (Boyer 1954) but has not gained commercial traction. Spinning methods are considered bottom-up approaches to plant protein texturization because anisotropic ingredients are formed first and then assembled into an end product.
Electrospinning applies high voltage and dry spinning to biopolymer solutions pushed through a needle or spinneret, forming nano-sized fibers. Ideally, proteins should be unfolded before electrospinning while avoiding insoluble aggregate formation. Electrospinning has been explored for various food-grade proteins (Nieuwland et al. 2013), including pea (Kutzli et al. 2019a & 2019b), common bean (Aguilar-Vazquez et al. 2020), and zein (Mattice et al. 2020). Overall, spinning technology for plant proteins is viewed much the same way as 3D printing—although the desired structures form, process throughput is too low for commercial scalability. Spinning also sometimes requires large amounts of water or organic solvents. As a result, there is ample room to optimize fiber formation through spinning technology or to explore combining these methods with other, more scalable methods. (See GFI’s solution for texturization innovations to learn more.)
Biological manufacturing methods
This section distinguishes enzymatic treatments from fermentation treatments. The former implies the addition of exogenous enzymes, and the latter is defined as a process that relies on enzymes produced in situ by a microorganism.
Enzyme treatment
As mentioned, transglutaminase can promote protein gelation, which affects end product texture and can be added before or after mechanical processes such as shear cell texturization (Manski, van der Goot, & Boom 2007). One study applied extrusion followed by enzymatic hydrolysis of soy protein (Ma et al. 2018). Extrusion combined with enzyme hydrolysis sharply increased the number of disulfide bonds and thus decreased thiol reactive groups. The soy protein’s water-holding capacity and solubility increased with increased extrusion temperature and hydrolysis time. Enzymes, therefore, can tune the texturization process of plant proteins to more closely recapitulate the functional attributes of conventional meat proteins.
Fermentation
Traditional fermentation of extruded plant-based products can alter their functionality (Maung et al. 2020). Planterra and MycoTechnology partnered to create OZO brand products using pea and rice protein fermented by shiitake mycelia. Fermentation improves the taste and aroma and enhances the pea and rice proteins’ liquid-holding capacity and texture. GFI grantee BZ Goldberg is applying fermentation to improve the flavor of plant-based meat. The ingredient optimization deep dive further discusses fermentation of plant proteins. For more information on fermentation used in alternative meat, see GFI’s science of fermentation page.
Post-texturization formulation
After texturization, plant-based meat may undergo hydration, marinating, breading, or other cooking and coating procedures. HMMA (wet TVP) typically contains fat and water, but marination can enhance fat content or incorporate additional components, especially those that are too sensitive to withstand extrusion. Dry TVP can be combined with hot water, broth, fats, or marinades that incorporate flavors and other functional additives, such as emulsifiers, to prevent separation. Additionally, the product can be coated, which could be a multi-step process with an adhesive component to affix additional ingredients such as spices, flours, and bread crumbs.
Some challenges arise after texturization. For example, if film-forming polysaccharides are present, the resulting protein could have lower breading and oil pickup during cooking (Yeater et al. 2017). The authors of this study note that plant protein powders can have these carbohydrate residues at adverse levels if the protein content is too low. Another study noted that high initial moisture content, protein denaturation, and starch gelatinization could contribute to low levels of oil transfer during frying (Rahimi et al. 2019). While infusion of plant-based meat with marinades can be complex, some techniques can optimize product manufacturing. For instance, adding freeze-thaw steps before marination can improve the diffusion of flavors and fats (Giezen et al. 2013; Kyriakopoulou et al. 2021).
Clearly, an abundance of research remains to be done to optimize multiple aspects of formulation and manufacturing.