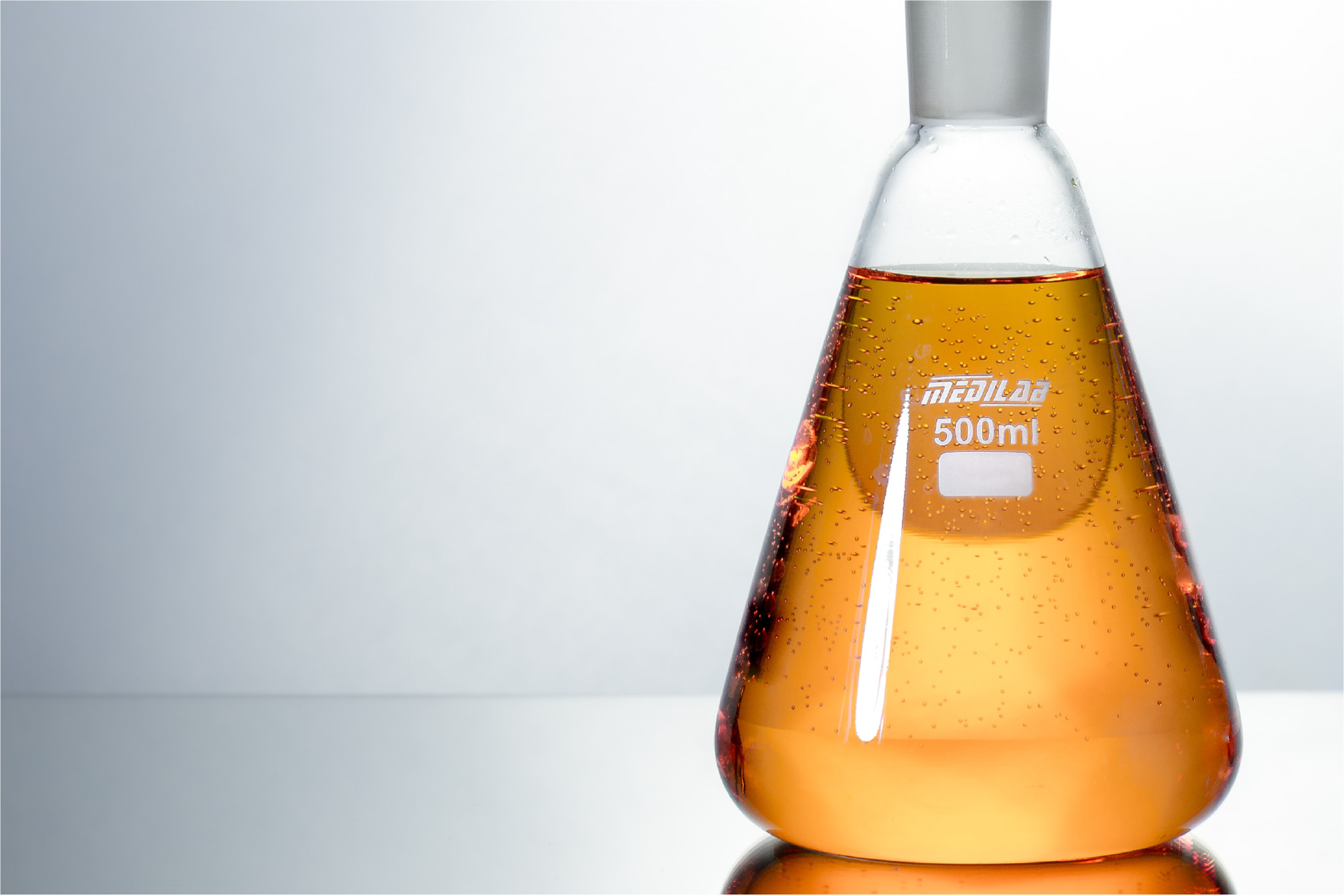
Deep dive: Cultivated meat cell culture media
Learn more about cell culture media used in cultivated meat production.
Cultivated meat cell culture media, part A
Introduction
Growing cells ex vivo requires the same fundamental inputs as required in vivo: a mixture of a carbon-based energy source (e.g. glucose), amino acids, salts, vitamins, water, and other components to support cell viability and vitality. This mixture, known as the cell culture medium, is the most important factor in cell culture technology. Although cell culture is routinely performed in academic labs and industrial bioprocesses, creating the biomass required for cultivated meat to achieve mass-market penetration at competitive prices will demand significant reductions in costs, innovations for serum removal, and optimization across a diverse set of species and cell types. An overview of cell culture medium composition and the factors at play to achieve price parity with conventional meat are discussed below.
Common components of cell culture medium
The first instance of culturing tissues outside of the body came from Sydney Ringer in 1882. By creating a balanced salt solution with similar pH, osmolarity, and salt concentration to that of an animal’s body, Ringer was able to keep various animal tissues alive outside of the body for several days. Subsequent work in the following decades first demonstrated that culturing cells in the presence of blood plasma (i.e. serum) or embryonic extracts assisted in cellular proliferation and viability, allowing tissues to survive for longer periods of time. Over time, researchers identified the importance of glucose, amino acids, glutathione, insulin, and vitamins in the sera being used (Yao and Asayama 2017). Once this was known, scientists aimed at uncovering the additional unknown essential components of serum and other extracts that permitted cell proliferation and viability.
In the 1940s and 50s, working with the first immortalized cell lines such as L cells (Earle et al. 1943) and HeLa (discussed in Cell Lines), scientists used iterative approaches to discover that low molecular weight dialyzed fractions of serum containing amino acids were necessary for cell survival. In 1955, Harry Eagle developed a Minimum Essential Medium by testing the amino acid requirements on several different cell lines, discovering that thirteen were indispensable. Eagle’s minimum essential medium additionally consists of glucose, six inorganic salts, eight water-soluble vitamins, and dialyzed serum. Variations on this medium were then derived using a variety of different cell lines as well as trial and error approaches that aimed at replacing serum with chemically defined components. These variations, including Dulbecco’s Modified Eagle Medium (DMEM), Iscove’s Modified DMEM, Ham’s F12, Medium 199, RPMI 1640, Leibovitz’s L-15, and others, still make up the majority of what are referred to as basal cell culture media in use for culturing the variety of cell types used today (Yao and Asayama 2017; Arora 2019).
What makes these formulations essential? Although formulations have been varied and optimized over time, the principal components of basal cell culture media have remained largely unchanged. Importantly, these variations may be cell-type specific, including for the cell types used in cultivated meat (described in Cell Lines). Therefore, rather than discussing optimal conditions for a specific cell line or species, only the general roles of each component of common basal media including glucose, amino acids, inorganic salts, vitamins, and buffers are briefly discussed below.
Glucose
Glucose (specifically D-glucose) is the most common energy input used in cell culture, although some media formulations use galactose or a combination of glucose and its metabolite, pyruvate. Industrially, it is produced enzymatically using amylase enzymes to breakdown starches from maize, potato, wheat, and other crops into constituent sugars used in various downstream products such as industrialized food, fermentation processes, or in this case, culturing of cells. Glucose enters the cell via transporter proteins on the cell surface, using either passive transport down its concentration gradient (more common) or ATP-dependent active transport. Once inside the cell, it serves as a reducing agent against oxidative stress in the form of NADPH generation via the pentose phosphate pathway, as well as a primary source of energy in the form of ATP generation via glycolysis.
In cell culture, glucose is used at concentrations between 5.5 and 55 mM, where the lower end is more common and similar to fasting blood glucose levels in humans. Different cell types will require different amounts of glucose. During periods of rapid cell proliferation and growth, as typically maintained during bioprocessing, glucose metabolism is high and can yield lactic acid even in the presence of sufficient oxygen, leading to pH changes (Zagari et al. 2013). Thus, glucose utilization and lactic acid levels are commonly measured and tightly controlled throughout a bioprocess (discussed in Bioprocess Design).
Amino acids
Amino acids are necessary to create proteins and other low molecular weight compounds such as nucleotides and small peptides. Amino acids can be split into two groups: essential and non-essential. Non-essential amino acids (NEAAs) can be synthesized de novo by an animal, whereas essential amino acids (EAAs) must be obtained through the diet. Generally speaking, pathways for the de novo synthesis of NEAAs are conserved in vertebrate species (Hou, Yin, and Wu 2015). In humans and many other animals, the EAAs include histidine, isoleucine, leucine, lysine, methionine, phenylalanine, threonine, tryptophan, and valine. NEAAs include alanine, arginine, asparagine, aspartate, cysteine, glutamate, glutamine, glycine, proline, serine, taurine, and tyrosine. However, EAA requirements can vary between species. For instance, dogs, cows, and pigs have the same EAA requirements as humans plus arginine, whereas cats and chickens require the same EAA as the former plus taurine and glycine, respectively.
Importantly, what is considered to be “essential” in cell culture is different than what is considered “essential” to a whole organism, as the diversity of cell types that may synthesize certain amino acids in vivo are not present in vitro (Figure 1). For instance, Eagle’s Minimum Essential Medium formulation lists 13 (L-enantiomer) amino acids as being essential across multiple cell lines in vitro: arginine, cysteine, glutamine, histidine, isoleucine, leucine, lysine, methionine, phenylalanine, threonine, tryptophan, tyrosine, and valine. As an example, arginine is essential in vitro as its biosynthesis in vivo primarily occurs between epithelial cells in the gut and proximal tubule cells of the kidney. Thus, arginine must be supplied in the absence of these cell types. Media that are particularly nutrient-rich (eg. DMEM/F12 or Medium 199) may contain all amino acids. Alternatively, NEAAs can be supplemented independently.
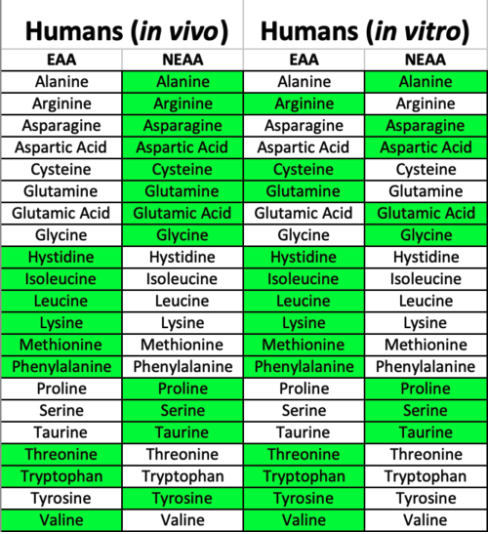
Figure 1. Essential and nonessential amino acids in vivo vs. in vitro varies due to some biosynthetic pathways being predominantly driven in specific cell types that are absent in vitro. Thus, additional amino acids become essential in cell culture.
Industrialized production of amino acids can be obtained through bulk extraction from protein hydrolysates (discussed later), chemical synthesis, or microbial fermentation and purification, with the latter being the most common (D’Este, Alvarado-Morales, and Angelidaki 2018). Amino acids enter the cell through a variety of transporter proteins on the cell surface, at rates influenced by the cell’s state and consumption rates due to protein production levels, cell cycle state, and other parameters. Once inside the cell, amino acids serve as substrates for many biosynthetic pathways and optimal concentrations are important for maintaining metabolic equilibrium. The majority of carbon mass in proliferative cells is derived from bulk amino acids rather than glucose or L-glutamine, despite these being the most rapidly metabolized (Figure 3, Hosios et al. 2016).
Ultimately, the levels of amino acids required for cell culture are determined not only by their utilization by the growing cells, but also by individual amino acid solubility, stability, and interaction with other medium components such as metal cations, all of which can change once in a complex mixture (Salazar, Keusgen, and von Hagen 2016). Consideration for all of these variables is highly complex and a full understanding of amino acid behavior, utilization, and optimization in a bioprocess has yet to be accomplished. Given the variety of biosynthetic pathways that involve amino acids, it is likely that amino acid content, concentration, and perfusion rate (when applicable) will need to be optimized for a particular bioprocess across species and cell types for parameters such as growth rates or protein content in the final product. Computational approaches to model specific utilization rates of amino acids and other basal media components are an active area of research (Quiroga-Campano, Panoskaltsis, and Mantalaris 2018) (discussed later).
L-glutamine
L-glutamine deserves special consideration as one of the most important amino acids included in cell culture media, as it is readily transported into cells and becomes a major contributor to protein biomass. It is a notable precursor of carbon and nitrogen-containing biomolecules such as the intermediate molecules used in the synthesis of other amino acids and nucleotides (DeBerardinis and Cheng 2010) and it can be added at concentrations 3-40x higher than other amino acids in the medium (Yao and Asayama 2017). During times of high cellular growth and proliferation, the demand for glutamine outpaces its supply, making it de facto an essential amino acid that can be readily metabolized as a replenishing alternative energy source (i.e. anaplerosis). At physiological pH in a cell culture medium solution, L-glutamine is unstable, resulting in its decomposition into pyroglutamate and ammonia, the latter of which is toxic to cells. Ammonia, therefore, is a tightly monitored and regulated metabolite in large scale bioprocesses that involve high densities of cells undergoing rapid growth (discussed in Bioprocess Design).
In order to avoid some of these disadvantages of L-glutamine, glutamate — which is more stable in solution — can be substituted in when working with cells expressing high levels of glutamine synthetase, an enzyme that enables intracellular conversion of glutamate to glutamine while consuming ammonia in the process. A more common practice involves supplementation with L-glutamine as a stable dipeptide in the form of alanyl-glutamine (i.e. GlutaMAX) or glycyl-glutamine, which enable cells to endogenously cleave the dipeptide for more controlled usage of the amino acids in the dipeptide. There is still much to learn about amino acid metabolism in cell culture. For instance, recent discoveries suggest L-glutamine is entirely dispensable for the culture of pluripotent stem cells (Vardhana et al. 2019).
Inorganic salts
The inclusion of inorganic salts is important in establishing and maintaining the osmolarity of the cell with its surrounding cell culture medium solution as well as serving as enzymatic cofactors and important components of receptor and extracellular matrix proteins. These inorganic salts are composed of cations and anions that fully dissociate in solution. The original minimal essential medium solution contained six inorganic salts (calcium chloride, potassium chloride, magnesium sulfate, sodium chloride, sodium phosphate, and sodium bicarbonate), which are based on Earle’s salt solution. Other formulations include additional inorganic salts containing zinc, copper, and iron, which have particular importance for a variety of cellular functions (discussed later).
Although all cells maintain a resting membrane potential, excitable cells such as neurons and skeletal muscle cells are particularly sensitive to changes in ionic concentrations that can readily affect their functionality and viability. Several basal medium formulations have thus been optimized for salt concentrations for neuronal (Bardy et al. 2015) and skeletal muscle cell culture that more accurately recapitulate the interstitial fluids surrounding these cell types. The osmolality or measurement of osmotic pressure within the medium is typically between 260 to 320 mOSM/kg (milliosmoles per kg of solute), although this can vary with cell lines that are particularly robust in varying solute concentrations such as insect cells (Rubio et al. 2019). Changes in the salt concentration, either abruptly due to medium changing or slowly due to water evaporation, can lead to osmotic shock. Thus, maintenance of osmolarity is an important component of cell culture.
Vitamins
Vitamins are classes of organic compounds that serve as a critical component for the maintenance and growth of cells. Most vitamins are essential in that they need to be obtained directly from the diet or cell culture medium with few exceptions (e.g. vitamin D synthesized by fibroblasts and keratinocytes of the skin or some B vitamins produced in low levels by intestinal microbiota). Vitamins are classified as either fat-soluble or water-soluble and can serve broadly as enzymatic cofactors, antioxidants, and hormones. Vitamins are processed in a variety of ways in vivo following ingestion, often in a complex sequence that ends in absorption into intestinal cells via membrane surface transporters. This complex sequence involved in absorption can be largely avoided in vitro, as hostile environments (e.g. stomach acid) or barriers (e.g. the blood-brain-barrier) are absent (Said 2011). Thus, vitamins are typically included in a medium formulation as a single chemical compound that can be processed and absorbed directly by cells in vitro.
Vitamins can also effectively function as a group of compounds (i.e. vitamers) where each compound can serve the vitamin’s functional role, albeit with varying properties. The natural production of vitamins in microbes and plants has made industrial production of vitamins via microbial fermentation possible, however, improvements in metabolic engineering strategies are needed to increase yields and sustainability in the industry. For these reasons, some vitamins are produced more efficiently via chemical synthesis (Acevedo-Rocha et al. 2019).
Water-soluble vitamins including riboflavin (vitamin B2), nicotinamide (vitamin B3), pantothenic acid (vitamin B5), pyrodoxine and pyridoxal (vitamin B6), biotin (vitamin B7), i-inositol (vitamin B8), folic acid (vitamin B9), cyanocobalamin (vitamin B12), and choline are typically added to and “essential” in cell culture media, sometimes in various modified forms in order to provide stability. Fat-soluble vitamins A, D, E, and K are excluded in basal medium formulations but can be added if necessary when dissolved in an organic solvent. Similar to the different in vivo versus in vitro requirements of amino acids, fat-soluble vitamins play specific roles for certain cell types or bodily functions and are thus only “essential” when culturing a relevant cell type. For instance, a metabolite of vitamin A, retinoic acid, is an important developmental morphogen (discussed in detail later) and may be included as an additive in media to derive spinal motor neuron cells from pluripotent stem cells (Patani 2016). Special consideration for vitamin stability must be taken when using serum-free medium formulations (discussed later) as the lack of stabilizing serum proteins can lead to rapid degradation via light, heat, oxidation, or pH fluctuations (Schnellbaecher et al. 2019). These properties make it advisable to reconstitute powdered B vitamins immediately before use (discussed later).
Buffering systems
Buffers are essential to cell culture systems as they serve to maintain pH at a constant level (for mammalian cells, generally 7.4 ± 0.4) despite changes in the composition of acids or bases that would otherwise alter the pH of the cell culture medium. Buffers are mixtures of a weak acid and its conjugate base or a weak base and its conjugate acid, where each mixture serves as a sponge to soak up free protons or hydroxide ions in solution, minimizing their effect on overall pH (Figure 2). Buffer systems in cell culture typically consist of either CO2-bicarbonate systems or buffering agents such as HEPES. As discussed in Bioprocess Design, a CO2-bicarbonate system can be achieved by exogenous addition of 5-10% gaseous CO2 (often delivered in bioreactor systems via sparging), which reaches equilibrium in solution with bicarbonate ions, forming a natural buffer system.
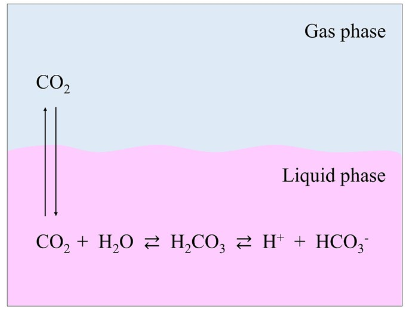
Figure 2. The pH control mechanism of culture media, based on the bicarbonate buffer system and the Henderson–Hasselbalch equation. When dissolved in water, sodium bicarbonate (NaHCO3) dissociates to form a sodium ion (Na+) and a bicarbonate ion (HCO3−). The latter reacts with H+ in solution to form carbonic acid (H2CO3), which dissociates into CO2 and H2O. These two reactions attain their respective equilibria. The CO2 in solution also reaches equilibrium with CO2 in the gas phase. As a result, increasing the concentration of gas phase CO2 increases the amount of CO2 that is dissolved in the culture medium, in turn raising the H2CO3 concentration and lowering the pH. In contrast, if the concentration of the gas phase CO2 is lowered, then the pH rises due to the reverse reaction. The relationship between the culture medium pH and the concentrations of CO2 and NaHCO3 can be expressed by the Henderson–Hasselbalch equation: pH=pKa+log[HCO3−]/[CO2] in liquid phase, where: pKa is the negative log of the acid dissociation constant. From Yao and Asayama, 2017.
pH slowly changes over time due to the respiration of cells and the release of additional CO2, which forms carbonic acid in solution, in addition to the metabolism of glucose and the formation of lactic acid. The resultant decreasing pH changes are counteracted by the inclusion of sodium bicarbonate in the basal medium itself. Importantly, added sodium bicarbonate should be proportional to the atmospheric CO2 being used to maintain equilibrium. For instance, for media containing 1.5 to 2.2 g/L sodium bicarbonate, 5% CO2 is recommended, whereas 10% CO2 is recommended for media containing 3.7 g/L sodium bicarbonate.
HEPES is a zwitterionic buffer that can be used in cell culture systems as a supplemental buffer, especially in the absence of CO2 exposure. As one of Good’s buffers, its high solubility, low toxicity, and membrane impermeability have made it attractive for use in cell culture applications. In the scale-up of highly proliferative stem cell populations, dissolved CO2 due to high metabolism can reach levels that are deleterious for cell growth and nutrient utilization (Kimura and Miller 1996). Attempts have thus been made to limit dissolved CO2 by culturing cells in the presence of atmospheric CO2 levels with added buffering capacity from HEPES or other Good’s buffers (Brodsky et al. 2013). This strategy may be useful for future scale-up efforts in cultivated meat. Consideration for the cost of the buffer must also be weighed, as it may constitute the most expensive component of a basal media formulation at scale (Specht 2018).
Media preparation
Out of convenience, most academic and lab-scale cell culture is performed using commercially available premade liquid media. However, large volumes necessitate on-site preparation of liquid cell culture media from reconstituted powdered medium ingredients. Powdered medium is more efficiently transported and stored, resulting in cost savings and reduced degradation of fragile ingredients (e.g. B vitamins), although recent product launches such as Gibco BenchStable permit shelf-stable basal medium formulations such as DMEM for up to 12 months if light exposure is avoided. Ideally, a powdered medium contains all of the components to be utilized and is created through a process known as micronization, where the average size of crystallized particles in the mix is reduced in order to increase solubility and homogeneity. When ready to use, the powder is typically reconstituted in a dedicated tank using high-quality water prepared by reverse osmosis, deionization, and filtration. The reconstituted medium is then itself sterilized by filtration (e.g. through a 0.22 µm filter), irradiation, or other methods discussed in Bioprocess Design (e.g. pulsed electric fields). The use of sterilization involving high heat is precluded by some heat-labile ingredients that may be part of the formulation. Other preparation methods for additional ingredients are discussed throughout.
Serum
As previously mentioned, a basal medium formulation is often sufficient to keep cells alive for short periods of time, but in order for them to proliferate efficiently over extended periods of time, a variety of animal sera (e.g. fetal bovine serum, horse serum, and others) and extracts (e.g. chick embryo extract) have historically been used. Notably, on a volumetric basis, serum-free formulations are now more dominant in their usage although FBS is still often included in routine cell culture in many academic settings and in some industrial settings. Serum is a high protein-containing mixture that contains growth and attachment factors, hormones, antioxidants, lipids, and other components (all described later) that mimic a proliferative, fetal-like state. Indeed, most sera used in cell culture are derived from fetal animals, which are rich in the necessary components and contain low immunoglobulin and complement content due to developmentally immature immune systems. As fetal bovine serum (FBS) is the most common sera used in cell culture, it will be used as a reference example throughout this section.
Originally employed in the late 1950s (Puck, Cieciura, and Robinson 1958), FBS has become a mainstay in biomedical research because it can supplement the growth of virtually all common human, animal, and even insect cell lines. As an added supplement for many cell culture applications in amounts typically 5-20% of total medium volume, FBS — when used — is often the most expensive part of performing cell culture.
FBS is harvested from a fetal calf any time during the last two-thirds of gestation following the discovery of pregnant cows due for slaughter. It has been estimated that up to 8% of cows in the slaughter line may be pregnant, making FBS a byproduct of the meat processing industry (van der Valk et al. 2018). It is prepared by the sterile collection of fetal blood followed by coagulation at low temperatures and centrifugation to remove clotting factors and blood cells. The serum supernatant is then filtered and assessed for a variety of quality controls including residual microbial or viral contamination, endotoxin, immunoglobulin content, and total protein, before being bottled and sold commercially, at prices exceeding $1000 USD per liter (at time of writing, July 2019) depending on quality control parameters (some described later), which vary by industry and use-case.
Despite its long history of use, FBS has several well-described issues that have made its replacement a priority in recent years. First, FBS contains hundreds or even thousands of different components and the true composition and amounts of these components are unknown, making it a chemically undefined product. The composition also varies by geographic region where a cow’s diet can vary, by batch within the same geographic region, by seasonality of collection, by the quantity and identity of antibiotics or hormones received by the mother, and by the gestational age of the fetus. Variability can also stem from a single bottled product originating from fetuses of different sexes (Elhofy, n.d.). This variability has led to a growing concern over serum’s contribution to irreproducibility of in vitro experiments within and between labs around the world (Baker 2016). Rigorous quality control involving testing of serum batches across multiple cell lines or experiments prior to purchasing a specific, well-performing large batch is often performed in industry but can remain burdensome from a labor and economic perspective for smaller academic labs. Thus, the inherent variability and undefined nature of FBS use leads to compounding external costs in quality control testing, experimental irreproducibility or conflicting results, and follow-up research to dissect irreproducible signals.
Second, FBS is a potential source of contamination from multiple organisms, including Mycoplasma, viruses, and bovine spongiform encephalopathy. Mycoplasma are a class of parasitic bacteria that lead to metabolic and gene expression variations for infected cell lines. Mycoplasma are likely the most common cell line contaminant, with recent estimates showing 11% of cell lines being infected, and rates as high as 70% in geographical regions where testing is not routine (Olarerin-George and Hogenesch 2015). Although presently FBS is routinely filtered using 0.1 micron systems that should theoretically capture Mycoplasma, suppliers cannot make this guarantee. The common cell line contaminants M. arginini and A. laidlawii, in particular, have been linked in origin to FBS, and ongoing cross-contamination of cell lines has likely propagated this contamination in laboratories since the 1960s and 1970s when FBS batches were routinely positive for these bacteria (Drexler and Uphoff 2002). Additional methods to decontaminate serum from Mycoplasma include gamma irradiation, however, this can also damage growth factors and other proteins in the serum (Baker 2016). Thus, the use of FBS is responsible for a non-trivial amount of bacterial contamination in cell lines today, leading to compounding problems concerning reproducibility and potential unknown variability stemming from some decontamination practices.
In addition to bacterial contamination, the threat of adventitious viral agents in FBS also persists. Regulations under USDA and the EU mandate the testing and/or treatment (via heat or irradiation) of eight viruses known to be present in FBS from all geographical regions of origin (Hawkes 2015). Although modern production methods make the risk of contamination in a validated batch low, viral contamination is often still detectable in batches that manufacturer screens claim to be negative (Gagnieur et al. 2014). Similarly, the threat of FBS containing the causative prion proteins involved in bovine spongiform encephalopathy (i.e. Mad Cow Disease, which manifests in humans as variant Creutzfeldt-Jakob Disease) is persistent and requires additional testing as well as documented traceability for the FBS origin. For instance, countries such as the USA, New Zealand, and Australia have no documented cases of bovine spongiform encephalopathy; thus FBS originating from these countries may be considered ‘safer,’ often commanding significantly higher prices and collectively comprises up to 90% of the serum supply for commercial therapeutics (Schnitzler et al. 2015). This fact has also incentivized fraudulent activity in the field, where manufacturers may opt for fake labels from New Zealand in order to solicit higher prices (van der Valk et al. 2018). Industry associations have formed in an attempt to mitigate these concerns. Nevertheless, the inherent risk of contamination from FBS poses threats to experimental and bioprocess reproducibility, drives price fluctuations, and can even incentivize bad actors that value profit over safety. Contamination will be discussed further from a food safety perspective in forthcoming deep dives.
Third, there is a limited global supply of FBS and there exists competition for it from profitable, mature industries. For instance, while the vaccine and biologics industries have begun to move to serum-free formulations (discussed later), the rise of cell therapies and stem cell research more generally has ushered in an impending demand that exceeds current availability. FBS is still included in some upstream manufacturing and expansion processes for cell therapies. Because FBS is a byproduct of a more lucrative product per animal (i.e. meat and dairy) and profits are retained by slaughterhouses rather than farmers, farmers have little incentive to increase cattle herds to meet a future FBS demand (Brindley et al. 2012). It has thus been hypothesized that “peak serum” has been met, with serum availability relatively stagnant and serum demand increasing dramatically as cell therapies begin to be approved (Brindley et al. 2012). Indeed, the prices of FBS have already begun to increase 300% over the past few years. The replacement of serum thus may be driven first by limited total availability followed by cost concerns that will spur replacement innovation in the field as non-pharmaceutical players are priced out. In the case of cell-based meat, this cost concern is already prohibitive, making FBS an economic nonstarter as meat products cannot be justified at prices that rival a cell-based therapeutic (currently at a cost of goods of approximately $50,000 and selling price of hundreds of thousands of dollars).
Lastly, the use of FBS carries ethical concerns, making its use inherently misaligned with one of the fundamental benefits of cultivated meat: animal welfare. A single liter of serum requires one to three fetuses, with roughly two million fetal calves used in serum collection annually, totaling approximately 800,000 liters of FBS produced per year. The collection process involves removal of the fetus from the mother’s womb and aseptic collection of blood by a syringe placed directly into the beating heart as this contains unclotted blood, raising concerns that the fetus could consciously experience the event as painful (van der Valk et al. 2018). Thus, the search for serum-free formulations (discussed later) is in alignment with the cultivated meat industry and general animal welfare concerns, manifested by replacement, reduction, or refinement of animal experiments or animal-based products in science.
View references featured in part A
Cultivated meat cell culture media, part B
Serum composition
As described above, serum is a high protein-containing mixture derived from blood that supports cellular growth and proliferation. Fetal bovine serum (FBS) is the most commonly used animal serum supplement in cell culture. But what exactly does FBS contain that makes it a near-universal cell culture supplement worthy of its high cost and known issues and how can understanding these components assist in creating replacements? As previously mentioned, the true composition of FBS is variable and unknown. It is out of the scope of the discussion here to speculate on the function of each component that may play different roles across the range of species and cell types used in cultivated meat. Therefore, similar to the approach for basal media described above, only the general roles of some components including hormones, key proteins, lipids, growth factors, and trace elements are discussed below.
Hormones
Hormones are a class of endocrine signaling molecules that come in various structural forms (e.g. protein, steroid). They mediate communication between distant tissues, traveling via the circulatory system. Hormones therefore are found in serum, which is unsurprisingly enriched with pregnancy-related hormones when harvested from a fetal calf. Common hormones listed as components in FBS include insulin, cortisol, growth hormone, parathyroid hormone, triiodothyronine (T3), thyroxine (T4), thyroid-stimulating hormone, follicle-stimulating hormone, testosterone, progesterone, prolactin, and luteinizing hormone. Most hormones mediate their effects by binding receptors located on the cell surface or in the cytoplasm, where they kickstart a signaling cascade that can regulate gene expression, metabolism, and growth, amongst other effects. However, some hormones only affect specific cell types. For instance, it is unlikely that follicle-stimulating hormone has any effect on a majority of cultured cells (excluding cell lines derived from ovary or testis).
Experiments designed to discover essential components of FBS via addition of singular components alone or in combination quickly revealed the essential role of insulin in cell culture, which regulates the intracellular transport of glucose and glucose metabolism. Thyroid-derived hormones can also influence cellular growth and proliferation programs in various tissues primarily by regulating key transcription factors governing these pathways or expression of growth factors such as insulin growth factor 1 (IGF1) (Näntö-Salonen et al. 1993). Other hormones can be added exogenously as needed. For example, corticosteroids such as dexamethasone may be added as soluble complexes directly to a cell culture medium or recombinant growth hormone can be used to boost cell proliferation and maturation. Notably, hormone-depleted FBS can be obtained by charcoal stripping if desired. With the exception of insulin, many hormones that appear in FBS are not commonly added to serum-free medium formulations and their actions are instead replaced directly by the downstream growth factors that they regulate (discussed later).
Albumin, fetuin, transferrin
FBS contains a high concentration of protein, typically reported as 30 – 45 milligrams per milliliter. The proteins albumin, transferrin, and fetuin, found in FBS, have particularly important roles in cell culture. Albumin is the most abundant protein in FBS (~50-60% of total protein content) and primarily serves as a carrier or stabilizer of a variety of different small molecules, vitamins, fatty acids, cholesterol, and metal ions (e.g. zinc, calcium, magnesium, manganese, cobalt, and nickel), which are able to directly bind to the protein at multiple locations (Francis 2010). It exhibits antioxidant properties by protecting molecules from oxidation or preventing them from participating in oxidation reactions. This is especially important in bioreactor environments where high metabolic rates, dissolved oxygen, and transition metals (e.g. cuprous and ferrous ions) can yield damaging reactive oxygen species. Albumin has also been shown to reduce the effects of shear stress (discussed in Bioprocess Design) on cells in bioreactors, although the mechanism is poorly understood (Francis 2010). Although not absolutely required, albumin is an important component of cell culture mediums and recombinant forms of albumin are frequently supplemented in serum-free formulations (discussed later).
Fetuin-A is a carrier protein synthesized and secreted by the liver and is one of the most abundant proteins in FBS (up to ~20 mg/mL) (Kundranda et al. 2005). In vivo, the protein primarily serves to bind calcium and phosphate in order to prevent ectopic calcification; however, it has since been understood to have a variety of functions (Nangami et al. 2013). For instance, fetuin-A is considered to be an attachment and spreading factor for cells, playing an important role for cell attachment to various substrates in vitro and allowing them to spread on a 2D surface, likely mediated by its role in triggering PI3kinase/Akt signaling pathways involved in proliferation (Sakwe et al. 2010; Kundranda et al. 2005). It also functions as an antagonist to transforming growth factor beta (TGFβ) signaling, a common growth factor used in some stem cell media (Szweras et al. 2002). Fetuin-A is considered to be dispensable for serum-free formulations but its attachment and proliferation-inducing properties may make it attractive for cultivated meat researchers. Indeed, other attachment factors found in FBS such as fibronectin, vitronectin, and collagen, are all commonly used as purified substrates in cell culture applications.
Transferrins are a group of proteins that assist in the control, transport, and delivery of ferric iron (Fe3+) to cells (serum transferrin is specifically discussed here) (Baker, Anderson, and Baker 2003). Iron is involved in many cellular processes, serving as a cofactor for proteins and enzymes involved in metabolism, DNA synthesis and repair, and heme-mediated oxygen transport in vivo (Puig et al. 2017). Its levels within a cell must be tightly regulated, as deficiencies can lead to apoptosis and a surplus can lead to toxic build-up of reactive oxygen species produced via the Fenton reaction (Thomas et al. 2009). Transferrins mediate this regulation with two ultra-high affinity ferric ion binding pockets. As consecutive iron ions are bound, the affinity for the transferrin receptor increases. This permits two iron-bound transferrins to form a complex with their receptor, resulting in the delivery of the iron content to intracellular carriers such as ferritin. Iron-free transferrin (i.e. apotransferrin) can then be recycled to resume scavenging free iron in the serum or cell culture medium. FBS is a rich source of iron and protein-complexed iron, which increases in concentration throughout gestation (Kakuta et al. 1997). In the absence of serum, ferric and/or ferrous iron is typically delivered as a sulfate or nitrate salt in the basal media, and recombinant transferrin is added to regulate iron homeostasis and scavenge free iron to prevent oxidative damage.
Lipids
Lipids are a broad class of compounds that are soluble in non-polar solvents. The lipids relevant to cell culture include fatty acids and their derivatives (e.g. phospholipids), sterols (e.g. cholesterol), fat-soluble vitamins (discussed previously), and glycerides (i.e. the stored form of fatty acids). Similar to its protein content, the lipid content of FBS varies in terms of both what molecules are present and their ratios. Some lipid molecules are still poorly understood in terms of what their role in vitro may be due to the highly complex interactions between multiple serum components. Key lipid components of FBS are described below.
Fatty acids are composed of a carboxylic acid head and a long unbranched hydrocarbon tail of varying lengths. They can be either saturated or unsaturated depending on the presence (unsaturated) or absence (saturated) of carbon-carbon double bonds. In addition to serving as the building blocks of stored fat, they can be used to target proteins to membranes, form membrane phospholipids and glycolipids, and derive other messengers such as hormones and the eicosanoids. The vast majority (>99%) of circulating fatty acids in vivo are bound to protein carriers such as serum albumin and enter cells via mechanisms still under investigation (Figure 2). It is believed that fatty acids dissociate from albumin at slow but defined rates and unbound fatty acids are taken up into the cell via passive diffusion or facilitated transport using specialized transporter proteins (e.g. CD36, FABPpm, FATP1-6) on the cell surface before becoming bound to cytoplasmic fatty acid protein carriers (e.g. FABPc) for downstream uses (Schwenk et al. 2010). Under physiological albumin concentrations, this dissociation and uptake occur as a saturable function of the unbound fatty acid, but at lower concentrations, the delivery is dependent on the albumin concentration (Sorrentino et al. 1989; Alsabeeh et al. 2018).
Serum albumin serves a similar role for carrying the fatty acid content in FBS, which contains a rich but variable amount of saturated and unsaturated fatty acids. However, unlike amino acids, the vast majority of fatty acids can be synthesized from glycolytic precursors. This synthesis in vitro relies on the addition of biotin (vitamin B7) to the cell culture media. Only two fatty acids are considered to be essential in humans and most other animals: alpha-linolenic acid (ALA, omega-3 fatty acid) and linoleic acid (omega-6 fatty acid) (Figure 3, Morimoto et al. 2005). A conundrum, therefore, is that it has long been observed that some cell lines in vitro are able to grow and fully function in serum-free medium formulations that completely lack all fatty acids and albumin, as evidenced by the lipid-free RPMI-1640 basal medium that is commonly used in cancer cell line culture (Whitford and Manwarin.; Wu and Näär 2019). Although this may be attributable to the metabolic changes in cancer cell lines, it is broadly thought that the addition of serum or added cocktails of fatty acids can lessen the biosynthetic load and boost cell proliferation, performance, or other parameters, but are not necessarily essential for most in vitro cell culture applications. In some cell lines (e.g. hybridomas), additional additive precursors to lipids such as ethanolamine are required (Murakami et al. 1982).
In general, however, fatty acid requirements are dependent on cell line and type and will likely need to be empirically determined for optimized cultivated meat production. Lipid-depleted FBS can be purchased following charcoal stripping or organic solvent precipitation to aid in determining experimental requirements (Alsabeeh et al. 2018). Many possibilities also exist for tuning the fatty acid profile of cultured skeletal muscle and adipocytes for nutritional purposes based on the fatty acids delivered in the cell culture medium or by genetic engineering strategies focused on metabolism.
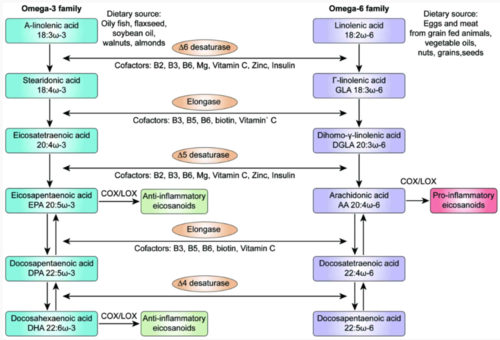
Figure 3. Dietary alpha-linolenic acid and linolenic acid are metabolized into a variety of signaling molecules known as eicosanoids. Tsoukalas et al. 2018.
Cholesterol
Cholesterol is a sterol lipid that serves as an important structural component of the plasma membrane, as well as a precursor to steroid hormones and vitamin D. Vertebrate species are able to synthesize cholesterol de novo but other animals such as arthropods and nematodes cannot. In the cell, it is distributed in different ratios amongst different membrane compartments (~30% of the plasma membrane is cholesterol) and serves to reduce membrane permeability and adjust membrane rigidity. In addition to cholesterol biosynthesis, cells can obtain cholesterol by receptor-mediated endocytosis of cholesterol-carrying lipoproteins in the serum. Lipoproteins allow hydrophobic compounds such as cholesterol and triglycerides to be transported through water or serum surrounded by hydrophilic proteins called apolipoproteins. Lipoproteins are colloquially recognized by their density and size (e.g. high-density lipoprotein (HDL), low-density lipoprotein (LDL)), although both contain cholesterol. In FBS, cholesterol is abundant (~300 µg/mL) and contained within lipoprotein complexes and delivered to cells in vitro as described. Similarly to fatty acids, the ability of cells to synthesize cholesterol makes it a non-essential ingredient; however, the addition of cholesterol may lessen metabolic load to improve upon other cell performance parameters.
Growth factors
Growth factors are a broad class of signaling molecules that stimulate growth, proliferation, and differentiation by initiating signaling cascades following binding to their respective receptors. They are arguably the most important reason for the success of FBS as a near-universal growth supplement. Some growth factors (e.g. hormones) have already been described. Here and in future sections, we highlight protein-based growth factors that can be produced via recombinant methods. Limited public information exists surrounding the complete individual protein makeup of FBS due to the sheer number of proteins and their variable amounts (Zheng et al. 2006). In comparison to proteins such as albumin and fetuin, growth factors are typically found in concentrations up to one million times less (ng/mL range), making them difficult to accurately quantify or detect. These quantities may actually be higher than found in adult bodies, as the fetal environment is enriched for growth stimulation. The few available reports indicate that typical growth factors found in FBS include members of the insulin growth factor family, fibroblast growth factor family, transforming growth factor family, and neuregulin family (Zheng et al. 2006). The role of some specific proteins from these families will be elaborated on in later sections.
Heparin
Heparin is a glycosaminoglycan produced in immune mast cells. It is most known for its role as an anticoagulant in vivo via its binding and activation of antithrombin III, leading to downstream anti-clotting effects. However, the evolutionary conservation of heparin in invertebrates that lack clotting systems such as shrimp, lobsters, and clams, suggests additional roles for heparin (Medeiros et al. 2000). Although some invertebrate heparins retain anticoagulant properties when used in mammals, it is speculated that its role may otherwise be in modulating immune function (Medeiros et al. 2000).
In stem cell culture applications, heparin binds to members of the fibroblast growth factor family (FGF), as well as some members of the platelet-derived growth factor (PDGF) and vascular endothelial growth factor (VEGF) families (Spivak-Kroizman et al. 1994; Andrae et al. 2013). This binding can have stimulatory or inhibitory effects on cell proliferation depending on cell type and which growth factors are included in a medium formulation. Additionally, heparan sulfate proteoglycans, which are structurally similar to heparin, can bind and sequester growth factors in the extracellular matrix (discussed in Scaffolding). Therefore, heparin in FBS-containing media may have a complex influence on stem cell proliferation due to its interaction with growth factors. Heparin’s potential inclusion in serum-free formulations used for cultivated meat will likely be dependent on the cell type and specific growth factors included in the media formulation.
Trace elements (minerals)
Minerals are chemical elements that have a biochemical function in the cell (Figure 4). The six inorganic salts included in Eagle’s minimal essential media discussed previously in part A contain calcium, potassium, magnesium, phosphorus, and sodium elements, generally considered to be the five major minerals in the human body. The other elements (outside of oxygen, carbon, hydrogen, and nitrogen) with biochemical roles in the body are detectable in small amount and make up the trace elements. These include sulfur, iron, chlorine, chromium, cobalt, copper, zinc, manganese, molybdenum, iodine, and selenium. Trace elements have a variety of important functions in cells or specific tissues, ranging from hormone production and function to cofactors for enzymes in amino acid, glucose, and lipid metabolism. Cells can be highly sensitive to levels of trace elements, as low amounts can lead to deficiencies and high amounts are often toxic. The roles of and requirements for major and trace elements are generally conserved across the species relevant for cultivated meat and seafood. These trace minerals can be readily obtained in cell culture by supplementing with FBS (Bryan et al. 2011), where they exist in a compound or complexed form rather than as a pure element (for instance, cobalt is obtained as cobalamin, the chemical name of vitamin B12). Trace elements will remain an important component of serum-free formulations (discussed later).
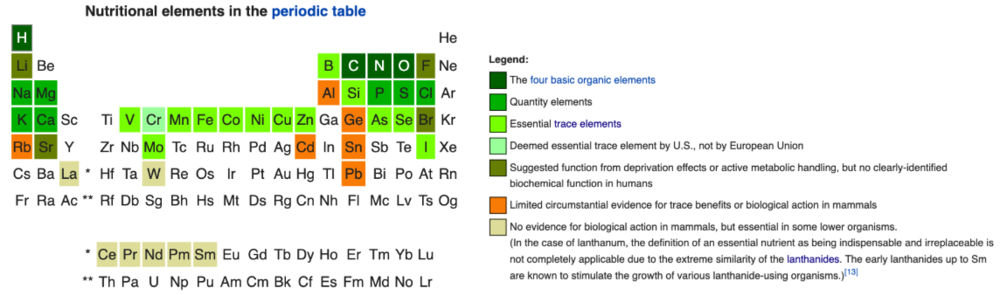
Selenium and glutathione
Selenium was discovered to be an essential trace element component of FBS during experiments in the 1970s (Yao and Asayama 2017). Selenium serves as a cofactor for evolutionarily conserved enzymes such as glutathione peroxidase and thioredoxin reductase, enzymes that regulate oxidative stress via reduction of hydrogen peroxide and thioredoxin, respectively. In serum, it is incorporated into glutathione peroxidase and other selenoproteins that contain a selenium-containing cysteine analog called selenocysteine in their structure. Selenoprotein P is one of the most important serum selenoproteins and carries up to 50% of serum selenium (Tujebajeva, Harney, and Berry 2000). Selenium can be taken in by cells as inorganic selenium via anion transporters, as selenoamino acids via amino acid transporters, or complexed to selenoprotein P via receptor-mediated endocytosis (Nickel et al. 2009; Burk and Hill 2009). In serum-free formulations, selenium is typically delivered as sodium selenite as part of a combined insulin-transferrin-selenium supplement (discussed later).
Glutathione peroxidase catalyzes the reduction of hydrogen peroxide and lipid peroxides using selenocysteine-containing glutathione as a supplier of electrons for the redox reaction (Arteel and Sies 2001). Additionally, glutathione serves as a reductive substrate in the regenerative pathway of vitamin C (Foyer and Noctor 2011). Thus, glutathione is an important cellular antioxidant. It can be biosynthesized by most animal cells, is water-soluble, and is found abundantly in its reduced and mixed disulfide forms in FBS, although it is not always an essential component for maintaining cell growth (Bump and Reed 1977).
Extracellular vesicles
Extracellular vesicles are membrane-coated vesicular structures released from most, if not all, cell types, including some types of bacteria, fungi, and plants. They are heterogeneous in size, generally between 10nm – 1µm and are derived from the endosomal system (i.e. exosomes) or shed from the plasma membrane (i.e. microvesicles). They contain cargo such as nucleic acids, proteins, and lipids, and are excreted into biological fluids, making them an important form of cellular communication (van Niel, D’Angelo, and Raposo 2018). Extracellular vesicles are found in high amounts in FBS and have complex and variable compositions of cargo with variable effects on different cell types. This adds an important additional dimension of complexity when considering variability due to FBS use.
Extracellular vesicles can be isolated from a fluid using ultracentrifugation or other filtration and capture technologies (Li et al. 2017), resulting in exosome-depleted FBS. Compared to normal FBS, exosome-depleted FBS can result in reduced cell growth across many cell lines (Eitan et al. 2015) and altered proliferation and differentiation in skeletal muscle cells (Aswad, Jalabert, and Rome 2016). The addition of the FBS-derived extracellular vesicles alone can restore some of these phenotypes, demonstrating their importance in cell culture (Eitan et al. 2015). Understanding the cargo of extracellular vesicles and how its components affect specific cell types may, therefore, be useful to pursue in cultivated meat applications such as assisting proliferation, differentiation, or maturation (Forterre et al. 2014).
Conventional examples of existing serum replacements
There are many more specific components of FBS that could be described in more detail; however, the former discussion covers the majority of the important FBS components, with consideration for those that may be considered for inclusion in a serum-free formulation. A wide range of efforts to replace serum have been tried and accomplished and many of these replacements are used today, permitting serum-free cell culture for a variety of applications. However, serum-free formulations will need to be further optimized specifically for cultivated meat.
The next section will explore a subset of the existing serum replacement strategies that have been conceived of or are actively being utilized in stem cell culture. These are merely a range of examples that serve to increase the understanding of what some current serum replacements look like and what they can teach us about novel replacement strategies. A discussion of these examples does not imply that they would be more or less suitable for cultivated meat versus other existing FBS alternatives.
Platelet lysates
Platelets are components of the blood that assist in clotting, wound healing, and general immune function. Platelets harbor a variety of different chemokines, growth factors, and adhesion proteins that can be released via lysis. Because of their abundance in the blood (in humans; 1.5 – 4.5 x 10^5/µL) and attainability through standard protocols for concentrating platelets following apheresis, human platelet lysates have been used for in vitro cell propagation since the 1980s. Platelet lysates contain a rich protein-heavy mixture of growth factors, proteins such as albumin (20-36 mg/mL), albumin’s typical cargo of vitamins, minerals, and lipids, immunoglobulins, as well as glucose and cholesterol. Therefore, platelet lysates are highly similar to FBS but have higher immunoglobulin content and may contain coagulation factors such as fibrinogen when produced without activation using thrombin (Burnouf et al. 2016).
Platelet lysates are obtained by concentration of platelets using various methods, followed by lysis via repeated freeze-thaw cycles, activation with thrombin or calcium chloride, bursting via sonication, or solvent and detergent treatments. It is currently unclear how upstream methods of lysate preparation relate to optimized downstream performance, but even so, many studies using primarily human mesenchymal stem cells report improved performance of platelet lysates vs. FBS across multiple criteria (Figure 8, Burnouf et al. 2016). For instance, substituting FBS with platelet lysates has been shown to significantly reduce population doubling times (Ben Azouna et al. 2012) and increase total outgrowth over multiple doublings (Schallmoser et al. 2007) in human mesenchymal stem cells. These effects have been replicated in other stem cell types, including skeletal myoblasts (Saury et al. 2018), and platelet lysates support general cell growth in a similar manner to FBS in many other stem cell types (Burnouf et al. 2016). It is perhaps not surprising that human cells perform well in human-derived platelet lysates and this improved performance may make platelet lysates potentially attractive for cultivated meat applications.
Platelet lysates offer potential advantages over FBS but several questions remain, including how human platelet lysates perform in non-human cells (obtaining platelet lysates from a specific animal species is likely to be economically nonviable for reasons described below and misaligned with animal welfare). Other areas of active research include understanding the role of high immunoglobulin A, G, and M content in platelet lysate, the optimal method of preparation, the role of donor blood type and age, and production, supply, and quality issues. Even if these were decidedly non-issues, the limited supply likely hampers its use in cultivated meat. Commercial distributors of human platelet lysate have been established in the USA and Europe. However, the current prices of human platelet lysate exceed $1000 per half-liter, making it more expensive than FBS. In theory, production capacity could scale and lower prices. For instance, it is estimated that 5-20% of platelet concentrates from whole blood donations expire during their 5-7 day storage limit and these could instead be frozen and used in cell culture applications, potentially opening up a 100,000 – 250,000L concentrated platelet byproduct industry globally, equal to roughly 25% of the global annual production of FBS (Burnouf et al. 2016). As this only takes into account wasted byproduct, this number could increase with demand fairly easily by more focused efforts on capturing platelet concentrates during blood donation and collection.
Another production method of platelet lysate is via iPSC-derived megakaryocytes, the immune cell that creates large numbers of platelets. Recent advances in differentiation to the megakaryocyte lineage makes it possible to create platelets in vitro that contain increased growth factor content compared to their in vivo counterparts (Strassel, Gachet, and Lanza 2018; Baigger et al. 2018). Despite this, it is hard to envision growing human cells to create a product to then be sold at an affordable price for the cultivated meat industry. Additionally, the high demand for platelets in human patients suggests any iPSC-derived platelet lysate product would first be sold at higher price points for clinical use, likely outbidding any reason for distributors to service the cultivated meat industry at lower prices.
Taken together, the upstream processing and quality control needed for safety, especially when pooling hundreds of donor samples in the case of blood-drawn platelets, likely make human platelet lysate economically nonviable for cultivated meat at scale. Nevertheless, an increased understanding of the unique composition of platelet lysates and growth factors contained therein can be leveraged for the creation of newer formulations produced using more economical methods (discussed later).
Conditioned media
Conditioned media is a term for the spent cell culture media following a period of cellular growth. It therefore includes all of the secreted factors that have accumulated in the medium over time, including growth factors, metabolites, cytokines, extracellular vesicles, and extracellular matrix proteins. The harvesting of conditioned media has been used for growing a variety of different stem cell types and even as a potential cell-free therapeutic (Pawitan 2014). The conditioned media from a specific cell type could be used to grow the same cell type or be used to grow an entirely different cell type altogether.
Conditioned media can also be used to triangulate growth factors required for cell growth. For instance, during the development of human embryonic stem cell cultures, the use of mouse embryonic fibroblasts as “feeder” cells was found to be sufficient to support the maintenance of pluripotency over time, and removal of fibroblast cells led to differentiation (Thomson et al. 1998). Studying the specific growth factors and cytokines secreted from these mouse embryonic fibroblasts led to the eventual development of the cell culture media formulations for pluripotent stem cells (Desai, Rambhia, and Gishto 2015). Today, mouse embryonic fibroblast-conditioned media is still sold as one option for pluripotent stem cell culture.
However, this methodology is not necessarily straightforward. The secretome of a single cell type such as myoblasts can contain hundreds of proteins, which may act independently or in concert to exert their effects (Henningsen et al. 2010). Additionally, some secreted factors may be inhibitory to desired processes such as cell growth and differentiation, and these may need to be removed entirely or kept at sufficiently low levels to prevent their effects (Csaszar et al. 2012). Significant work will be needed to understand the secretome from many of the understudied species and cell types used in cultivated meat production.
Nevertheless, similar strategies using conditioned media to grow cells for cultivated meat have been conceived. For example, the Japanese startup Integriculture has filed a patent for a system called CulNet, which describes a series of interconnected feeder cells that produce conditioned media to feed a different group of cells (羽生 and 川島 2018). A similar conditioned medium system has been filed by the cultivated meat company Fork and Goode (Forgacs and Gupta 2019). Such strategies may be sufficient to supplement cell growth and assist in the discovery of growth factor requirements for new cell lines, however, the affordability of growing multiple cell types as a means of supplementing cell growth versus simply producing the important growth factors through more cost-effective methods (discussed later) may be difficult to reconcile.
Single protein solutions: sericin
The use of a single protein that could stimulate cell growth and proliferation in a similar manner to FBS would be advantageous from a cost and usability perspective. Sericin is one of two major protein constituents of silk produced by the silkworm Bombyx mori. It is considered a byproduct of the silk textile industry, however, its unique amino acid composition, hydrophilicity, and biocompatibility yields potential use cases in the biological sciences and biomedicine (Aramwit, Siritientong, and Srichana 2012). In cell culture, the addition of sericin has assisted in the proliferation of various mammalian cell lines (Figure 5, Liu et al. 2016; Terada et al. 2002), attachment of mammalian cells (Tsubouchi et al. 2005), and spreading (Martínez-Mora et al. 2012), all of which are important qualities of FBS supplementation. These results have extended to other cell types such as myoblasts (Fujita et al. 2010) and species such as insect cells (Takahashi et al. 2005). Few studies examine in detail the mechanism of sericin’s proliferative qualities and further studies are needed to make these determinations. Nevertheless, the success of a single protein such as sericin holds promise for the discovery of other proteins with similar biological properties that could be used for FBS replacement in cultivated meat applications.
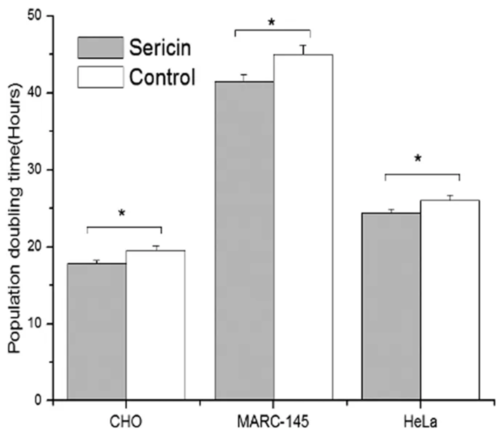
Figure 5. Medium supplemented with sericin (30 µg/mL) displayed improved population doubling times versus control (medium supplemented with 10% FBS) across 3 different cell lines. From Liu et al. 2016.
Serum replacement
Translational human embryonic stem cell research has dealt with many of the same issues related to FBS inclusion as the cultivated meat industry is currently tackling. Knockout Serum Replacement is one example of an attempt to replace FBS in a medium for a single cell type such as embryonic stem cells. Its composition is chemically undefined (discussed later) due to variable lipid content, but is relatively consistent from batch to batch. Its ingredient list includes 12 amino acids (both essential and non-essential), antioxidants (vitamin B1, reduced glutathione, vitamin C), 19 trace elements, and transferrin, insulin, and lipid-rich albumin (J, D, and Lynn 1998). When used with an appropriate basal medium formulation and added growth factors, knockout serum replacement can stimulate embryonic stem cell self-renewal and maintain pluripotency over time in a similar and often improved manner over FBS. Therefore, the inclusion of a specific set of ingredients found in FBS, many of which have been previously discussed, offers a sufficient strategy for FBS replacement and lays the groundwork for a discussion of the majority of work that has been done to replace FBS through the formulation of chemically defined media.
Forthcoming writing will cover the creation of serum-free medium formulations and various cost-reduction strategies necessary for commercializing cultivated meat.
View references featured in part B
Cultivated seafood cell culture media
Introduction
Although aquatic animal cell lines from embryonic and other lineages have been successfully established and studied, the field is in its infancy compared to cell culture from terrestrial vertebrates. In particular, a full-scale optimization of media composition has not been performed for any aquatic species (Chan et al. 2024), though substantial progress has been made recently. Here, previous cell culture studies from aquatic species are discussed in order to glean insights regarding what components are likely or unlikely to be necessary in an optimized, animal component-free formulation suitable for producing cultivated seafood. Comparisons of in vivo nutritional requirements and meat composition for aquatic and terrestrial species are also referenced, although due to potential limited translatability between in vivo and in vitro characteristics, such comparisons should be considered qualitative in nature.
Media composition is considered separately for fish embryonic stem (ES)-like cells, other fish cells, and cells from crustaceans and mollusks. The term ES-like cells is used because while some of the lines mentioned have been shown to express multiple ESC markers and to exhibit other stem cell-like characteristics such as forming embryoid bodies, others have been less fully characterized. ES-like cells should be understood to include both ESCs and other cells that may be partially differentiated.
Optimizing culture media for aquatic species is a tractable problem, made easier by the plethora of research already available from terrestrial species. However, much work is still needed to actually perform systematic optimizations of the concentrations of various components for teleost fish, crustaceans, and other aquatic taxa. Still further optimization will be required to fine-tune media composition for specific species and cell types.
Comparison of culture media for ES-like cells from fish and mammals
Compared to mammalian cell culture, much less is known about the in vitro requirements of fish cells. The most common media formulation used to grow fish ES-like cell lines was originally developed for medaka cells, a small fish commonly used as a research model organism (Hong, Winkler, and Schartl 1996). This formulation, called ESM1 in the original publication, includes not only fetal bovine serum (FBS) but also fish serum and fish embryo extract. Two recombinant human growth factors, FGF2 (also known as bFGF) and LIF, are included as well. A comparison of ESM1 and some of its derivatives with the serum-free Essential 8 (commonly known as E8) (Chen et al. 2011) and B8 (Kuo et al. 2020) formulas optimized for human ESC and iPSC culture reveals several similarities and differences (Table 1).
Table 1 features a comparison of E8, B8, and several media formulations used to culture fish ES-like cells. Components not separated by a horizontal line are those likely to substitute for one another, due to playing a similar function or interacting with similar receptors or signaling pathways. Bold borders indicate that the publication reported directly testing the effect of this component on fish cell growth and finding a positive effect (in the case of Nikkhah et al. (2023), where all components’ concentrations were optimized, borders are shown on only the three components with the strongest impact on growth rate). Double borders indicate components (besides basal media) shown to be essential, rather than simply growth-enhancing, although the essentiality of TGFβ is apparent only in long-term growth assays (Kuo et al. 2020). Color scales for β-mercaptoethanol, selenium, FGF2, FBS, and fish serum facilitate visualization of concentration differences between formulations. Question marks indicate that the publication did not state a concentration for the reported component. Note that the B8 media formulation uses the thermostable variant FGF2-G3, so its effective concentration in the media may be higher than it appears relative to other publications. Antibiotics and buffers are not shown. Also see Dash et al. (2010).
All formulations are based on common basal media such as DMEM, L-15, or a mixture of one or both of these with F12, and contain buffers, some form of antioxidant or reactive oxygen species scavenger, and a source of selenium ions. They contain either the same or similar recombinant growth factors. LIF, included in ESM1, and TGFβ/NODAL, included in E8, might play a similar role to one another, as it has been shown that treatment with TGFβ induces robust expression of LIF in glioblastoma cells (Peñuelas et al. 2009). Similarly, treatment of explants from the developing rat kidney with either LIF or TGFβ induced expression of LIF as well as a modest increase in expression of TGFβ (Plisov et al. 2001). NRG1, included only in B8, enhances growth but is not required (Kuo et al. 2020). E8 and B8 contain two components with no direct analog in ESM1: transferrin and insulin. In addition, E8 and B8 contain substantially higher concentrations of sodium selenite and FGF2 than ESM1. ESM1, unlike E8 and B8, contains FBS, fish serum, and fish embryo extract. These animal-derived components serve as sources of transferrin, insulin, selenium, and FGF2 (or analogs thereof), and thus at least some of these components will likely be required to produce optimized serum-free media.
What is not needed for culture of fish ES-like cells?
Most publications using fish ES-like cells employ media formulations based on ESM1. The only successful instance of serum-free culture of fish ES-like cells was in medaka, and was achieved by supplementing DMEM with IGF2 (Yuan and Hong 2017). This formulation, while able to support growth, did not perform as well as the control formulation, which contained all the same components as ESM1 except for LIF, and was not tested for its ability to support long-term culture. An essential step in identifying optimized media formulations for use in fish stem cell culture will therefore be the identification of the additional components of serum and embryo extract that are necessary and sufficient for optimal growth of fish stem cells in animal-free media. The composition of naturally-occurring serum is described in detail in part B above. Identification of a set of components that can support robust growth of fish ES-like cells in the absence of serum will require careful testing of a number of candidates and optimization of their respective concentrations.
Some components of ESM1 are frequently omitted in other studies, and therefore can probably be deprioritized in attempts to find optimized animal-free formulations. The growth factor LIF was used only during early passages in the original publication (Hong, Winkler, and Schartl 1996), and is one of the components most commonly omitted in other studies (Ho et al. 2014; Fan et al. 2017; Béjar, Hong, and Alvarez 2002; Chen et al. 2005; Kim et al. 2018; Parameswaran et al. 2007; Bryson et al. 2006); Nikkhah et al. 2023), suggesting that it may not be essential at least in some species or lines. In an ES-like line from Indian major carp, LIF was shown to stimulate growth and to help maintain an undifferentiated state, although both effects were quite modest when tested in the presence of other growth factors (Dash et al. 2010). ESM1 contains glutamine, pyruvate, and non-essential amino acids (NEAA) as additional nutrient sources, but cells from turbot (Chen et al. 2005), olive flounder (Kim et al. 2018), barramundi (Parameswaran et al. 2007), haddock (Bryson et al. 2006), and zebrafish (Nikkhah et al. 2023) have been successfully cultured without these supplements. Glutamine was also omitted in studies of ES-like cells from Japanese sea bass and red seabream (Chen et al. 2003; Chen, Sha, and Ye 2003), consistent with the glutamine independence of mammalian ESCs (Vardhana et al. 2019).
Two studies are notable outliers: ES-like cell lines from barramundi (Parameswaran et al. 2007) and haddock (Bryson et al. 2006) were both successfully cultured in L-15 with 15-30% FBS. However, systematic comparisons to media containing recombinant growth factors, fish serum, and embryo extract were not performed, so it cannot be concluded that L-15 with FBS was sufficient for optimal proliferation. Although elimination of FBS needs to be the eventual goal when it comes to cultivated meat and seafood, the fact that some fish ES-like cells from common food species will grow in the absence of fish serum or embryo extract is encouraging because it suggests that ES-like fish cells recognize and require the same growth factors that have already been well characterized for serum-free culture of non-aquatic cell lines. Unfortunately, the three ESM1 components that will be essential to replace for cultivated seafood applications are consistently used across all (FBS) or most (fish serum, fish embryo extract) studies of ES-like cells from food-relevant fish species.
Requirements for serum and growth factors
It is unclear to what extent the necessity of each of these components has been systematically tested in most of the above-mentioned studies. The importance of FGF2 and FBS in cod (Holen, Kausland, and Skjærven 2010) and of FGF2, FBS, and fish serum in turbot (Chen et al. 2005) were experimentally demonstrated by subtracting these individual components from the complete medium. The reverse strategy — adding specific components to a minimal medium — revealed that, for carp ES-like cells, fish embryo extract and FGF2 were the most capable of supporting growth when added individually to basal media containing NEAA and sodium pyruvate, while FBS, LIF, and fish serum had weaker effects (Dash et al. 2010). Interestingly, Nikkhah et al. (2023) identified PDGF as one of the factors with the strongest impact on the growth rate of zebrafish cells, with a substantially stronger effect than IGF1 or FGF2. Because this particular growth factor has not been consistently used in other studies of fish ES-like cells, its addition might represent a straightforward means of improving growth or reducing the need for serum relative to previous formulations.
Differences in growth factor concentration requirements between fish and terrestrial cells and between fish cells of different species may result not only from the intrinsic growth factor requirements of these cells but also from differences in growth factor stability at different temperatures (Chen et al. 2012). Even when considering a particular growth factor or family of growth factors, homologues from different species may have slightly different properties. In some cases, it may be important to match the species of the growth factor to the cells, for example by using fish growth factors to grow cultivated fish. Venkatesan et al. (2022) tested the ability of growth factors from a wide variety of vertebrate species to support the proliferation of mouse fibroblasts. Interestingly, they observed substantial species differences in the proliferation-promoting properties of certain growth factors, including examples where fish growth factors—such as tilapia IGF2 and salmon, tilapia, and medaka FGF2—outperformed those from other taxa. This suggests that species-specific growth factors may not always be the most desirable and that it may be beneficial to test growth factors from several taxa to find the best performers for a specific application.
Reduced-serum formulations for fish ES-like cells
Since serum is a complex mixture of proteins, peptides, and other molecules (discussed in parts A and B above), identifying the minimal set of components that can substitute for serum will be a key challenge. One interesting approach is the use of protein hydrolysates, extracts, or other undefined components as serum substitutes. Batish (2022) tested several different hydrolysates, including pea, mushroom, yeast, and algae, and found that hydrolysate-supplemented cultures of zebrafish ES-like cells could be grown with 1-2.5% serum with comparable growth rates to those grown in 10% serum. The research team has also filed a patent (Ovissipour et al. 2023) and published a follow-up study (Amirvaresi and Ovissipour 2024) related to this work. It is also noteworthy that higher concentrations of hydrolysate had detrimental effects on cell growth and viability, underscoring the need for careful optimization.
Similarly, Stojadinović et al. (2024) grew both zebrafish ES-like cells and Japanese quail myoblasts under reduced-serum conditions (1% in the case of the zebrafish cells) and tested a variety of algal and cyanobacterial extracts for their ability to substitute for the missing serum. Early results indicated that extracts of the cyanobacteria Arthrospira platensis and microalgae Dunaliella tertiolecta may be promising candidates.
Alternatively, serum can be replaced by a set of defined factors, such as growth factors, other proteins such as albumin and transferrin, lipids, and minerals. Nikkhah et al. (2023) performed a systematic optimization of defined growth media for zebrafish ES-like cells with the simultaneous goals of improving growth rate, minimizing cost, and minimizing the global warming potential (GWP) associated with the media ingredients. This project was focused on developing media for cultivated seafood and supported by a GFI grant. This study ultimately produced three media formulations that balance growth rate, cost, and GWP. One of these (formulation 3, see Table 1) used 7% FBS (compared to 10% in the baseline formulation) while reducing GWP to 65% of baseline, reducing media cost by 24%, and increasing growth rate by 51%.
However, in all these cases, serum-free growth was not achieved, indicating that—at least under the conditions tested—hydrolysates or other factors were able to substitute for part of the function of serum but not all of it. As mentioned earlier, Yuan and Hong (2017) were able to culture medaka ES-like cells for short periods under serum-free conditions with the addition of IGF2, though with reduced performance. While researchers have yet to fully crack the code to achieve fully serum-free and animal-component free media formulations that support robust, long-term growth, these early successes at serum reduction provide a useful roadmap. Future studies should consider combinations of hydrolysates or extracts with defined components to reduce the cost and environmental impacts of the media used for cultivated meat.
Below, several candidate serum components that could be necessary for optimal survival and proliferation of fish ES-like cells are discussed. Many of these are routinely used for serum-free culture of mammalian ESCs, and were discussed in further detail in part B of this series.
Role of insulin and insulin-like growth factors in fish
Although zebrafish have been demonstrated to respond to human insulin and to develop insulin resistance in a similar manner to mammals when exposed to high doses (Marín-Juez et al. 2014), insulin’s effects on fish stem cell proliferation are thus far unclear. However, the related insulin-like growth factors IGF1 and IGF2 have been better studied in this context. Moderate levels of IGF1 supplementation led to a modest increase in the growth of a fibroblast-like catfish cell line (Cyrino and Mulvaney 1999). Similarly, Nikkhah et al. (2023) observed a positive effect of IGF1 on the growth of zebrafish ES-like cells, though this effect was weaker than that of many of the other components tested. IGF1 showed only a very weak ability to stimulate proliferation and was unable to support the maintenance of an undifferentiated state in Indian major carp (Dash et al. 2010).
In contrast, one study demonstrated more profound effects using IGF2. The medaka ESC line HX1 showed a dramatic change in morphology and loss of expression of stem cell markers when transitioned from media similar in composition to ESM1, described above, to DMEM alone. Both of these effects were largely rescued when the cells were transitioned to DMEM containing IGF2 (Yuan and Hong 2017; Figure 6). Further, in the same study, DMEM with IGF2 was able to support derivation of blastomeres, although slightly less efficiently than control media. However, it is unclear whether this reflects a difference between IGF1 and IGF2 or a species difference.
Insulin, because of its demonstrated ability to support the growth of mammalian ESCs, and/or IGFs, because of their ability to improve survival and proliferation in fish, are likely to be necessary components of any serum replacement strategy. It is unclear to what extent insulin, IGF1, and IGF2 may be able to substitute for one another, since all three are known to interact with multiple receptors and to overlap in their receptor specificities, albeit with substantially different affinities (Griffeth, Bianda, and Nef 2014; Frasca et al. 1999; Andersen et al. 2017).
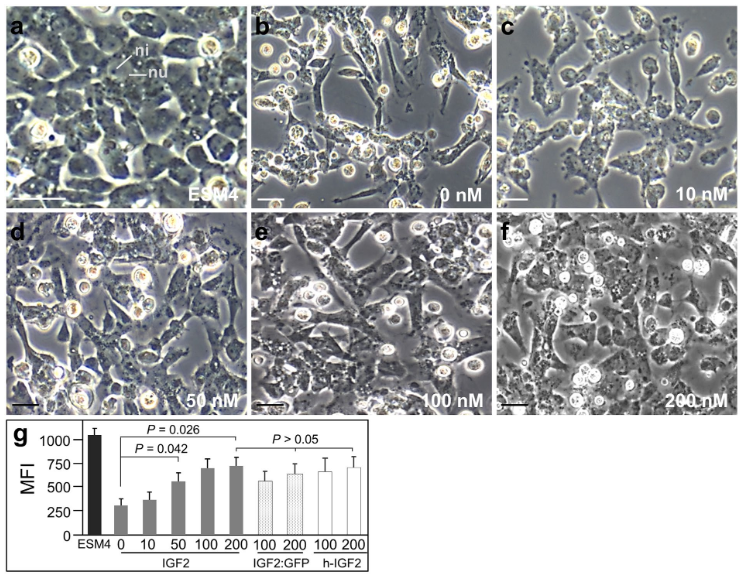
Role of transferrin and iron in fish
Transferrin is involved in the regulation of iron metabolism in both fish and other vertebrates by binding to free iron ions (discussed above in part B). This both prevents them from causing the formation of reactive oxygen species and simultaneously increases the availability of iron at appropriate sites within cells (Asmamaw 2016). As in other vertebrates, iron is an essential micronutrient in fish. The dietary iron requirement for Atlantic salmon was estimated as 60 mg iron per kg of diet, similar to the 50 mg/kg of diet recommended for beef cows, with iron-deficient salmon developing hypochromic microcytic anemia. It is important to bear in mind that these numbers are based on the whole organism and reflect the needs of many cell types. Raw Atlantic cod contains 0.38 mg of iron per 100 g serving, lower than raw steak, 1.85 mg per serving, or raw chicken, with 0.92 mg per serving in light meat and 1.22 mg per serving in dark meat. Other fish are more comparable to terrestrial species, such as raw skipjack tuna, 1.25 mg per serving.
Such quantitative differences in iron requirements or accumulation may reflect species differences in cellular requirements for iron, differences in how easily iron is absorbed, or some combination of these two factors. However, the fact that both iron and its carrier transferrin appear to behave similarly between fish and terrestrial vertebrates suggests that they may be similarly required in cell culture media, albeit possibly at different concentrations. In other words, the addition of transferrin to fish stem cell media is likely to be beneficial. However, when transferrin is used in media formulations, it is typically included at a concentration high enough to raise some concerns over its contribution to the production cost of cultivated meat. Therefore, alternative iron carriers that can substitute for transferrin, such as ferric citrate (Clincke et al. 2011; Neumannova et al. 1995), may be worth exploring. DMEM already contains iron in the form of ferric nitrite, but the optimization of the iron concentration specifically for culture of fish ES-like cells could also yield improved results.
Selenium requirements in fish
Selenium is present in E8 media at a concentration of 80 nM (Chen et al. 2011). This concentration may not be high enough for maximal growth; further optimization of E8 led to the B8 formulation, in which selenium is included at a concentration of 116 nM (Kuo et al. 2020). In contrast, concentrations between 2 nM and 8 nM have been used for culture of fish ES-like cells, and some studies use no defined selenium source at all (see Table 1). Nikkhah et al. (2023) found that selenium—along with PDGF and FBS—had a stronger impact on proliferation rates than the other components tested. Their optimized formulation contained a selenium concentration 7.4 times that of B8, further suggesting that selenium concentrations in prior studies may have been suboptimal. It is possible that cellular requirements for selenium are different between fish and mammals, or between fish species, so it is worth considering what is known about the requirements for selenium in fish.
Selenium requirements vary substantially across fish species, from 0.03 mg/kg of diet recommended for salmonids to 0.7 mg/kg of diet for juvenile grouper. The recommended minimum selenium dosage for beef cows is 0.1 mg/kg of diet, within the range recommended for various fish species. Fish also accumulate selenium within their muscles. The selenium content for raw Atlantic cod is higher, at 33.1 μg per 100 g serving, than that of raw steak, 21.1 μg per serving, or raw chicken, whether light meat, 17.8 μg per serving, or dark, 13.5 μg per serving. As with transferrin, there is no obvious reason why selenium would not be required for fish stem cell media in similar concentrations as for cells from terrestrial vertebrates. Identifying the optimum level for any given fish species should be part of any serum replacement strategy.
Choice of buffering agent
Standard practice in culturing fish ES-like cell lines is to maintain cultures under ambient CO2 conditions and to rely on HEPES as the primary buffering agent (discussed above in part A). One study found that chick ESC cultures were negatively impacted by the presence of HEPES or other zwitterionic buffers (Poole, Reilly, and Flint 1982), but it is unknown whether the same effects are likely in fish cells, and if so at what concentrations. Systematic testing of various buffering agents might be helpful in finding ideal culture conditions for fish ES-like cells.
Implications for serum-free culture of fish ES-like cells
The combination of factors that will allow for the replacement of serum and other animal-derived components for fish ES-like cells while supporting optimal proliferation rates, and their optimal concentrations, is currently unknown. A good starting point will be to test the components shown to be capable of replacing serum in terrestrial vertebrate cell culture: selenium, transferrin (potentially in conjunction with adjustment of iron concentrations), insulin, and various growth factors. IGF2 in particular is a strong candidate, as its addition to the media can support serum-free culture of medaka ESCs, albeit with a reduction in growth rate compared to conventional media (Yuan and Hong 2017). It is likely that the addition of other components together with IGF2 will allow for high and sustained proliferation rates of fish ES-like cell cultures without animal-derived ingredients.
Media for non-embryonic fish cells
In general, published media formulations for non-embryonic cells from fish are somewhat simpler than most of those discussed above, although they also typically contain FBS and suffer from a similar lack of systematic optimization. Primary cultures from muscle (presumably containing a mixture of myocytes, myosatellite cells, and other cell types) of rainbow trout and gilthead sea bream have been initiated using DMEM with 15% horse serum, and subsequently cultured in 10% FBS (Rescan et al. 1994; Montserrat et al. 2007; Castillo et al. 2002). Under these conditions, cells fused and eventually formed large myotubes over the course of 10 to 15 days (Montserrat et al. 2007; Castillo et al. 2002). More recently, Zhang et al. (2023) compared several basal media formulations in terms of their ability to support the proliferation of primary cells derived from large yellow croaker (Larimichthys crocea) muscle tissue. They found that F10 resulted in more rapid proliferation than DMEM/F12, which in turn outperformed DMEM (all formulations were supplemented with 10% FBS and 10 ng/mL FGF2). They also demonstrated that cells expanded in F10 could subsequently differentiate and fuse.
A continuous muscle cell line from the carp Labeo rohita maintained in L-15 grew best with 10% FBS and 10 ng/mL bFGF (Goswami et al. 2023). Another muscle line, this one from Atlantic mackerel (Scomber scombrus), was maintained in L-15 with 20% FBS and 1 ng/mL bFGF (Saad et al. 2023). A myogenic line from Asian seabass, also known as barramundi (Lates calcarifer) grew best in L-15 with 20% FBS, though the FBS concentration could be reduced to 5% with only a fairly modest decrease in growth rate (Pawaskar et al. 2025). Another myogenic line, this one from black sea bream (Acanthopagrus schlegelii), was cultured in DMEM with 20% FBS and 10 ng/mL bFGF (Han and Gong 2025). Reducing the FBS concentration to 15% or 10% had little effect on growth over the first two days of culture, but resulted in a modest decrease in growth rate and final cell numbers at later time points. A myofibroblast line from Australasian snapper (Chrysophrys auratus) grew best in M199 HBSS, though CIM, L-15 and MEM HBSS also supported cell proliferation (Chong et al. 2022). In the same study, proliferation increased with higher FBS concentrations up to 25%. IGF1 and IGF2 (at 100 ng/mL) both substantially increased proliferation, whereas bFGF and TGFb1 (at either 1 or 100 ng/mL) had a very modest impact and no impact, respectively.
Dong et al. (2023) demonstrated that Auxenochlorella pyrenoidosa extract (APE) could partially substitute for FBS in supporting the growth of cultured goldfish muscle cells, including in multi-passage experiments. Subsequent work by the same research group (Dong et al. 2024) showed that ascorbic acid (but not several other antioxidants) and the ITS-X supplement (consisting of insulin, transferrin, selenium, and ethanolamine) further increased cell proliferation when combined with APE. The combination of APE, ascorbic acid, and ITS-X resulted in further improvement, and was able to support growth of the cells under fully serum-free conditions. However, it is important to note that both studies also included fish muscle extract in their media formulations. APE’s growth-promoting activity most likely depended on the proteins present in the extract, as enzymatic hydrolysis abolished its effect (Dong et al. 2024). Metabolomics and proteomics experiments suggested candidate pathways that might be responsible for the growth-promoting effects of both FBS and APE, though experimental validation would be required to confirm the involvement of any given pathway.
Similarly, Lim et al. (2024) were able to successfully culture the above-mentioned mackerel myoblast line using a media formulation containing 2.5% FBS (compared to 20% in the original formulation). They found that rapeseed protein isolate—a byproduct of canola oil production—could partially rescue the reduction in growth under low-serum conditions. By adding insulin, sodium selenite, AA2P, linoleic acid, FGF2, TGFb, and transferrin, and by swapping the L-15 basal media for a 1:1 mixture of L-15 and F-12, they were able to achieve comparable growth to the 20% serum control. However, when serum was eliminated entirely, growth slowed dramatically even with these supplements. Interestingly, the initial screening phase of this project also indicated that Chlorella protein isolate was highly effective at improving growth under serum-reduced conditions. Similarly, a thesis project from the same lab was successful in growing bluefin tuna cells in serum-reduced but not serum-free media (Karimy 2024).
Zhang et al. (2024) developed a serum-free formulation able to support the growth of primary large yellow croaker myoblasts. Cell densities using the serum-free formulation were 65% of those of serum-containing controls, with the biggest difference between the conditions occurring on the first day of culture, and similar growth rates over the following two days, perhaps implying a difference in initial cell adhesion rather than growth. Although the formulation did contain bovine serum albumin (BSA), it represents an intermediate step toward fully animal-free media, since a defined component like albumin may ultimately be easier to replace. The researchers also found that hydrocortisone was an especially important component of the media under these conditions. More recently, Wei et al. (2025) developed a low-serum formulation for the same species.
A number of studies have also reported methods for the culture of adipogenic cells from various species of fish, as summarized by Sugii et al. (2022). L-15 was the most frequently used, though DMEM and DMEM/F12 were also common. Most studies’ formulations also included 10% FBS and antibiotics, often with additional salts, buffers, or glutamine. A recent study focused on large yellow croaker similarly used DMEM/F12 with 10% FBS for routine passaging of both satellite cells and adipose-derived stem cells, with the addition of 10 ng/mL bFGF for the initial cell isolation step (Zhou et al. 2025).
Fibroblast-like cells from the spiny red gurnard Chelidonichthys spinosus were successfully cultured over multiple passages in L-15 with 10% FBS (Shimada and Tsuruwaka 2024). A continuous cell line with an epithelial-like morphology isolated from southern bluefin tuna was successfully maintained over 8 months in L-15 containing 10-20% FBS (Bain et al. 2013). Concentrations of FBS below 10% resulted in reduced proliferation rates. Human FGF2 was included during isolation and early passages, but whether it affected survival or proliferation was not assessed.
Aside from the media formulation itself, the process by which cells are transitioned to serum-free conditions may play a role as well. Serum-free culture of ovarian cells from channel catfish (using UltraCulture SFM as the basal medium) has been achieved by gradual reduction in the serum concentration present in the media (Radošević et al. 2016). Although this cell type is not likely to be relevant to cultivated meat, the strategy of gradually adapting cells to serum-free conditions may be applicable to other cell types.
For example, Jozef et al. (2025) developed a serum-free formulation for use with a widely used cell line derived from the gills of rainbow trout. The formulation consists of L-15 basal media supplemented with ITS-X, BSA, a chemically-defined lipid mixture, a trace element mixture, biotin, vitamin B12, EGF, and hydrocortisone. When transitioning the cells to serum-free media, they compared the effects of a rapid transition (termed “sink or swim”) versus those of a gradual weaning process. They found that the weaning protocol was a quicker route (by two months) to a fully-adapted cell line and that the weaned cells were less prone to senescence than those transitioned using the sink or swim protocol. Lim et al. (2024) also demonstrated some positive benefit of an adaptation period when transitioning Mack1 cells to lower-serum conditions, though as discussed above, additional optimization of the formulation was also necessary.
Surprisingly, when Jozef et al. (2025) attempted to replace BSA with recombinant human albumin as a non-animal-derived alternative, they observed negative effects on cell proliferation and metabolic activity. This is consistent with the finding of Lim et al. (2024) that, while BSA performed somewhat well at rescuing growth under low-serum conditions, recombinant human albumin had an inhibitory effect on mackerel myoblast growth. Several explanations are possible here: First, the albumin protein sequences from humans and cows may inherently have different effects on cultured cells. The implications of this for cultivated meat and seafood would be quite minor since human protein sequences can be expected to be limited to use as research tools and not as food ingredients for consumer acceptance reasons regardless of their effectiveness. However, it might indicate that species differences between albumin proteins in general are likely to be important for performance in culture. Alternatively, the differences in performance might be attributable to some difference in the state of the recombinant and animal-derived albumin. This could be some negative factor in the recombinant albumin (indicating a need for improved purification methods), some positive factor complexed with animal-derived albumin, or a difference in phosphorylation or other post-translational modifications.
Importantly, it has been demonstrated experimentally that the addition of microplastics to culture media decreases cell attachment and proliferation in mackerel muscle cells (Sun et al. 2024). Depending on the quality of the water source used, it may be necessary for cultivated meat and seafood bioprocesses to implement additional testing and filtration steps to ensure that microplastics and similar contaminants are not present at harmful concentrations. This will be important not only for ensuring bioprocess efficiency but also for the safety of the final food product.
Amino acid requirements in fish
Amino acid requirements in terrestrial species were discussed in part A of this series. In vivo, the same amino acids are essential in most tested fish species (Wilson 2003) as in humans. The exception is arginine, which is essential in vivo in fish but only essential in vitro in human cells. Quantitative dietary amino acid requirements of a number of fish species may serve as useful guides for researchers attempting to optimize cell culture media for particular fish species, but it is unclear how direct the translation between quantitative in vivo and in vitro requirements will be. Amino acid requirements of various Mediterranean fish species (Tibaldi and Kaushik 2005) and salmonids (Lall and Anderson 2005) have been reviewed in detail.
Which amino acids, if any, are required in vitro but not in vivo in fish species is unknown. The successful growth of medaka ESCs in DMEM supplemented with only IGF2 (Yuan and Hong 2017) suggests that the in vitro essential amino acids are likely similar between fish and mammals, although this leaves room for differences in optimal concentrations. Amino acid concentration may be an important factor in species-specific optimization of media for both embryonic and non-embryonic fish cell lines, especially in cases where minimizing the cost of media is essential. However, it may not be necessary to achieve the goal of finding a media formulation that works reasonably well for culturing cells from most fish species.
Of note, zebrafish and some other fish species lack the gene for inositol-3-phosphate synthase. As a result, inositol has been considered as an essential nutrient in models of zebrafish metabolism (van Steijn et al. 2019), whereas it is non-essential in mammals. Myo-inositol has been identified as a dietary requirement for optimal growth in carp, tilapia, and parrot fish (Jiang et al. 2009; Khosravi et al. 2015; Shiau and Su 2005), although it is worth noting that only the latter species lacks the inositol-3-phosphate synthase gene. In the absence of exact measurements of the activity of these various homologues, it is worth keeping in mind that optimization of inositol levels may be an important step in developing optimized basal media for fish and that this optimization may need to be performed on a species-by-species basis.
Differentiation media for fish
Inducing cells to proliferate rapidly and efficiently is one important part of the puzzle, but it is equally important to identify suitable conditions to promote the differentiation of these stem cells into mature myofibers, adipocytes, and possibly other cell types.
Inducing differentiation of adult stem cell types such as satellite cells is often fairly straightforward. For example, Saad et al. (2023) induced their mackerel satellite cells to differentiate using a serum starvation protocol in which cells were grown with serum throughout the proliferation phase followed by the cessation of feeding. The use of serum starvation—a common method for triggering myogenesis—implies the use of serum, which is both incompatible with the economics of large-scale meat cultivation and associated with environmental, ethical, and health concerns. However, Messmer et al. (2022) were able to mimic the effects of serum starvation by examining the transcriptional changes in serum-starved bovine cells. By adding ligands to those receptors found to be upregulated during serum starvation, they found that the combination of transferrin, insulin, and lysophosphatidic acid was able to mimic the effects of serum starvation. Similar strategies could be employed to identify ideal serum-free differentiation media for fish adult stem cells. Identification of additional media additives that enhance differentiation or maturation may also lead to improved outcomes. For example, Ji et al. (2024) found that the addition of DHA to the differentiation medium (M199 with 1% horse serum) enhanced myogenesis in primary grass carp (Ctenopharyngodon idellus) myoblasts.
Adipogenesis is routinely initiated in cultured cells via a combination of insulin, dexamethasone, and 3-isobutyl-1-methylxanthine (IBMX), sometimes with additional other molecules (Sugii et al. 2022). For food safety reasons, it may be necessary to identify alternative strategies. Some progress has been made on this front, with the combination of rosiglitazone (a PPARγ agonist) and insulin shown to induce adipogenic differentiation without the need for dexamethasone or IBMX, including in serum-free media (Mitić et al. 2023). The serum-free version of the differentiation protocol—though not the serum-containing version—translated well across species (cow, sheep, pig, and mouse). It is unknown to what extent this cross-species translatability will extend to fish. Another promising approach, especially in fish, is the addition of fatty acids to the culture media (Sugii et al. 2022; Salmerón 2018). Interestingly, fish cells appear to show some level of phenotypic flexibility that allows myogenic cells to take on an adipocyte-like phenotype in response to media supplementation. Mackerel myogenic cells treated with insulin, dexamethasone, IBMX, and a lipid mixture accumulated fatty acids (Saad et al. 2023). Similarly, large yellow croaker satellite cells transdifferentiated toward an adipogenic-like fate when treated with horse serum and oleic acid (Wei et al. 2025). Protocols that rely primarily on lipid supplementation may have the additional advantage of making it easier to control the fatty acid profile of the final product, which will be important for both nutrition and flavor.
Pluripotent cells can be more difficult to differentiate into a specific target cell type due to the fact that they are not predisposed toward a specific lineage. Thus, it is necessary to provide these cells with specific cues to guide their differentiation process. Xu et al. (2013) identified several compounds that seem to promote myogenesis to varying extents in zebrafish iPSCs, including FGF2, three different GSK3β inhibitors, two calpain inhibitors, and the adenylate cyclase activator forskolin. Efforts to develop and optimize differentiation media for specific cell lines may be informed by this and similar studies as well as previous research into the molecular mechanisms underlying myogenesis and adipogenesis in fish (Bomkamp et al. 2023). Less-differentiated, multipotent adult cell types such as MSCs can be expected to fall in between unipotent and pluripotent cells in this regard.
Osmolality
Teleost fish maintain the osmolality of their extracellular fluids (Figure 7) within a fairly narrow range of around 300 mOsm/kg by actively either taking in (for freshwater fish) or excreting (for marine fish) salt (Kültz 2015). This is close to DMEM’s osmolality of between 310 and 360 mOsm/kg. It has been demonstrated that at least some fish cell lines show high osmotolerance, with an upper limit for acute exposure around 700 mOsm/kg (Gardell et al. 2014). Consistent with this, most of the fish cell culture studies cited above do not deliberately adjust osmolality, and media used to culture cells from Nile tilapia (Z. Fan et al. 2017), which is restricted to fresh or brackish water, is of a similar formulation to those used for cells from marine species.
Therefore, we can generally expect the correct target osmolality for cells from osmoregulator fish species—those that actively maintain the osmolality of their body fluids—to be relatively similar to that of mammalian cells. However, careful optimization of osmolality—along with other factors like CO2 concentration, temperature, and pH—can still be quite important for optimizing the performance of individual cell lines (Hodne et al. 2012).
The osmolality of the culture media will generally be an even more important factor in cells from fish that are osmoconformers, meaning that the osmolality of their extracellular fluids matches that of their environment. Osmoconformers are rare among aquatic vertebrates and include elasmobranchs and hagfish (Kültz 2015). Mesenchymal stem cells from shark embryos were successfully cultured in media with a lower solute concentration (in this case expressed as osmolarity) than adult cartilaginous fish plasma, an observation that may be related to the fact that the fluid within the egg contains a lower solute concentration than the surrounding seawater (Parton et al. 2007). The culture of cells from osmoconforming species may thus require explicit consideration of osmolality as it relates to both species and the life stage from which the cell line was derived.
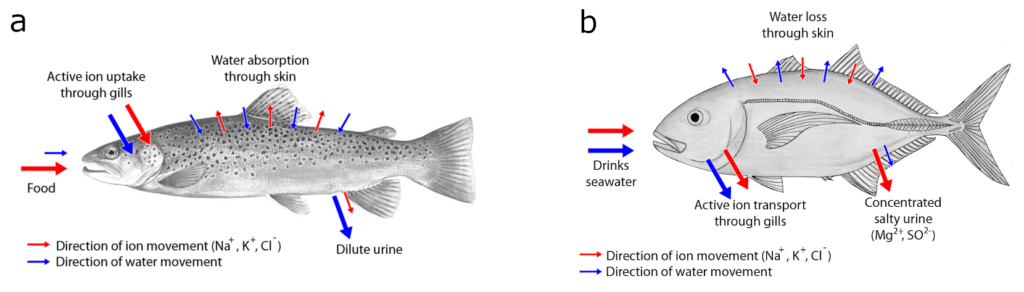
Media for crustacean cell culture
In general, the in vivo requirements for most minerals between fish and aquatic crustaceans are fairly similar, with some important differences (Davis and Gatlin 1996). Additionally, it may be possible to learn some lessons from insect cell culture, due to the close evolutionary relationship between these groups. As in vertebrates, selenium is thought to be required by shrimp and potentially other crustaceans, although a precise value for the requirement has not been reported. Although evidence for regulated transport and storage of iron has been observed in crustaceans, no evidence for iron deficiency in shrimp has been reported. This is consistent with the fact that crustaceans primarily rely on the copper-containing hemocyanins rather than iron-containing hemoglobin for oxygen transport. Indeed, most malacostracans (a group that contains both shrimp and crabs) lack hemoglobin entirely (Burmester 2015). Consistent with this, the dietary copper requirements for shrimp may be between 6-fold and 35-fold higher than those for fish, depending on the species (Davis and Gatlin 1996).
It is unclear whether crustaceans’ lower iron and higher copper requirements reflect only differences in oxygen transport mechanisms or if requirements of individual cells will also follow the same trend under culture conditions. Besides iron, the only minerals recommended for inclusion in feed formulations for fish but not for shrimp or lobster are iodine and manganese (Davis and Gatlin 1996). However, these are most likely required only for the proper functioning of particular organs or systems, rather than generally required by cells (whether fish or land animals), and are not included in the standard DMEM formulation. In summary, iron and copper deserve special attention when adapting media formulations from fish to crustaceans because of their vastly different roles in these organisms. However, oxygen transport, the role for which these minerals are best appreciated, works very differently in culture compared to in vivo, so it is unclear how well whole-organism dietary requirements will translate to requirements of single cells.
Many attempts have been made at culturing cells from shrimp, with some success, yet continuous cell lines have not yet been reported in the academic literature. However, the wealth of studies performed on primary cells provides some insights into what media formulations show promise (Ma, Zeng, and Lu 2017). In general, it seems that shrimp cells are adaptable to a variety of basal media formulations, but which is the most successful seems to be dependent on the cell type in question. Osmolalities used were higher than that of DMEM, ranging from 472 to 760 mOsm/kg. FBS has generally been found to support growth of shrimp cells, but may not be sufficient for optimal growth. Various extracts from shrimp or related species are often, but not always, helpful. FBS and chitosan both supported growth of cultures initiated from shrimp embryos, and a combination of FGF2 and IGF2 further stimulated proliferation (T.-J. Fan and Wang 2002). An extensive review of cell culture studies in shrimp has been published elsewhere (Ma, Zeng, and Lu 2017), and includes discussion of both basal media formulations as well as supplements such as FBS and various shrimp extracts.
Walsh et al. (2025) attempted to isolate ES-like cells from shrimp (Litopenaeus vannamei) using a variety of media formulations, all adjusted to an osmolarity of ~780 mOsm. The most successful formulation was based on 2X L-15 with added cholesterol and 20% FBS. Replacement of sodium with potassium had no effect, whereas LIF and bFGF had a positive impact on growth. These cells did not grow in formulations based on DMEM, M199, or filtered seawater. The effectiveness of Grace’s Insect Media in supporting shrimp cell growth was unclear due to the presence of thraustochytrid contaminants. In contrast, Sudarshan et al. (2023a) found that DMEM was effective in a study of a different shrimp species (Macrobrachium rosenbergii) for isolation and culture of embryonic cells. However, a continuous cell line was still not achieved. In this study, the medium was adjusted to 420 mOsm and supplemented with 10% FBS, antibiotics, and an antifungal.
A subsequent study by the same group (Sudarshan et al. 2023b) compared Macrobrachium rosenbergii cells grown in three different growth media and found that those grown in Opti-MEM had the shortest doubling time (0.92 days) over the first four days in culture, while DMEM:F12 (1.22 days) and DMEM high glucose (1.87 days) also supported growth to a lesser extent. They also compared the metabolites present in the three different cultures and in control media without cells and found that palmitate was consumed by cells cultured in Opti-MEM, but was produced by the cells in the other conditions. The authors suggested that the higher palmitate levels in Opti-MEM—enabling the cells to shift to a mode of net palmitate consumption—might have contributed to its improved performance. A third study by this group modified this protocol by initially seeding the cells using a DMEM-based formulation and then changing to the same OptiMEM-based formulation as before, which they found improved the initial attachment of the cells (Sudarshan et al. 2024).
Despite the challenges reported in establishing shrimp cell lines, the shrimp dumplings demonstrated by Shiok Meats in early 2019 and the whole shrimp demonstrated by CellMEAT in 2021 point to the feasibility of culturing shrimp cells to create a cultivated food product. CellMEAT also claims to have developed serum-free media compatible with their shrimp cells.
Primary cells from crab hepatopancreas were successfully cultured in 3x concentrated L-15 buffer reconstituted in artificial seawater (Sashikumar and Desai 2008). The viability of these cells was reduced in the presence of FBS or various crab extracts. However, these negative effects of FBS are likely cell type-dependent rather than species-dependent. Primary crab hemocytes grew well in 2x concentrated L-15 with amino acid-sugar supplement and 15% FBS (Sivakumar et al. 2019). Similarly, 2x L-15 with 10% FBS was successfully used to grow crab eyestalk neurons, whereas 5% FBS was found to be insufficient to support survival (Wajsenzon et al. 2016). A recent report (Jang et al. 2022) described the isolation of lobster primary muscle cells using 2x L15 medium (based on previous reports). Their results also suggested that the addition of either the molt hormone 20-hydroxyecdysone (up to 4 uM) or the juvenile hormone methyl farnesoate (up to 100 nM) could enhance proliferation. However, supplementation with either copper, iron, and zinc or the combination of both hormones did not appear to be effective. The role of the ecdysteroid hormones in regulating the proliferation and differentiation of crustacean cells was reviewed in detail by Musgrove et al. (2024).
As in other taxa, a more complete understanding of the in vivo roles of various growth factors in crustaceans would enable more deliberate hypothesis generation. This can then be followed up by experimental validation of the effects of specific growth factors or combinations thereof. For example, a recent analysis of receptor tyrosine kinase genes across crustacean, hexapod, and tardigrade species provides a framework for understanding the diversity of insulin, EGF, FGF, VEGF, and PDGF receptors within and across species (Flores et al. 2024). This knowledge can inform future media development efforts, for example by helping researchers to decide which isoforms of a given growth factor should be compared for their effects in a particular media formulation.
Media for molluscan cell cultures
Cell cultures from molluscs have similarly received little research attention. Primary cardiomyocytes from oysters were successfully isolated using shrimp cell culture medium (Jayesh et al. 2013), and could be maintained with or without FBS (which enhanced viability but was not essential) (Balakrishnan et al. 2024). Isolation of octopus muscle cells was achieved using osmolarity-adjusted L15, though long-term maintenance of these cultures was not described (Nesher et al. 2019). Recently, Qin et al. (2025) established a cell line from scallop (Chlamys farreri) larvae. They found that the cells could be grown in L-15 medium with a number of additives, including FeCl3, 5% FBS, scallop serum, and scallop ovarian extract. The addition of FBS and scallop serum extended the longevity of the cultures, but proliferation was not observed. Addition of FeCl3 or scallop ovarian extract caused the cells to proliferate, and a greater effect was observed when both were added together.
Effects of culture media on nutrition, flavor, and other end product properties
Besides those ingredients necessary for supporting the proliferation and differentiation of seafood cells in culture, efforts to develop media formulations for commercialized cultivated seafood should also consider the effects of media components on end product properties. Although some of these properties will arise from the attributes of the cells themselves, additional media ingredients might be added to improve the flavor, appearance, or nutritional properties of the product. Indeed, a recent survey of alternative meat and seafood companies found that the most common method by which omega-3 ingredients were added to cultivated products or prototypes was through the culture media. Omega-3 fatty acids, especially the long-chain varieties found in marine sources, are well-known for their nutritional benefits and also play a role in seafood flavor (Luo et al. 2024; Carneiro et al. 2022). Some of the same ingredients used in plant-based seafood (Luo et al. 2024; Coleman et al. 2022) or aquafeed (Stachowiak and Szulc 2021) might also be used as media components.
However, the relationship between the ingredients added and the composition of the final product may not always be straightforward, as both pre- and post-harvest metabolism can be expected to alter some compounds (Carneiro et al. 2022). This situation is highly analogous to that of conventional meat and seafood, where the composition of the final product results from the interplay between the animal’s diet and its biology.
Nyunoya et al. (2025) compared several fish cell lines’ ability to convert shorter-chain omega-3s (ALA; available from terrestrial plant sources) to longer-chain ones (EPA and DHA; primarily synthesized by marine microorganisms). Somewhat surprisingly, they found that lines from bluegill (listed as salmon in the publication due to an improperly authenticated cell line), trout, and two carp species were moderately or highly efficient at converting labeled ALA into EPA. The same lines converted EPA to DHA at low—but detectable—levels. Compared to the same-species cell line (derived from fin), primary carp hepatocytes showed worse performance in converting ALA to EPA, but better performance for the EPA to DHA conversion step. An eel hepatocyte line performed poorly in both respects, while pufferfish eye cells showed no conversion of ALA to EPA or of EPA to DHA, though they were fairly efficient at converting EPA to the DHA precursor DPA. The lack of ALA conversion was not unexpected, given that the tiger pufferfish genome lacks the genes for the relevant desaturase enzymes.
These differences—whether they reflect differences between species, cell types, individual cell lines, or some combination thereof—suggest that the omega-3 supplementation needs of different aquatic animal cell lines could be substantially different. If some cell lines can use ALA as a precursor in meaningful quantities, those lines’ need for supplementation with long-chain omega-3s might also be lower than one would otherwise assume. Assuming that fatty acid synthesis behavior is consistent across cell lines from a given species, we might hypothesize that trout, bluegill, and carp cells would require supplementation with ALA and DHA (with quantities dependent on the target fatty acid profile), but their need for external EPA might be minimal. Given their poor conversion efficiencies, eel and tiger pufferfish cells might need more comprehensive fatty acid supplementation. In all cases, such hypotheses represent only a starting point. It will be necessary to use empirical methods to develop media formulations optimized for individual species and cell lines that support efficient proliferation and differentiation while producing a final product with desirable organoleptic and nutritional properties.
Conclusion
Optimized, low-cost, animal component-free media formulations for use with seafood-relevant species are desperately needed in order to move cultivated seafood from a promising idea to an economically viable solution to the environmental, ethical, and food security problems associated with current methods of seafood production. Fortunately, there is a wealth of data on the nutritional needs of seafood species and their biological differences from common terrestrial species raised for meat. In addition, successful optimization projects for mammalian cells (Kuo et al. 2020) can provide a roadmap for those attempting similar goals in seafood species. Serum-free culture of medaka ESCs has been demonstrated (Yuan and Hong 2017), and more recent studies have demonstrated serum-reduced or serum-free culture of cells from a variety of food-relevant fish species. However, further optimization is needed to reduce costs, increase proliferation rates, replace animal-derived components such as albumin, and optimize formulations for additional species. These studies will take time and effort, but there is a clear path ahead toward the optimized media formulations needed to make cultivated seafood a reality.