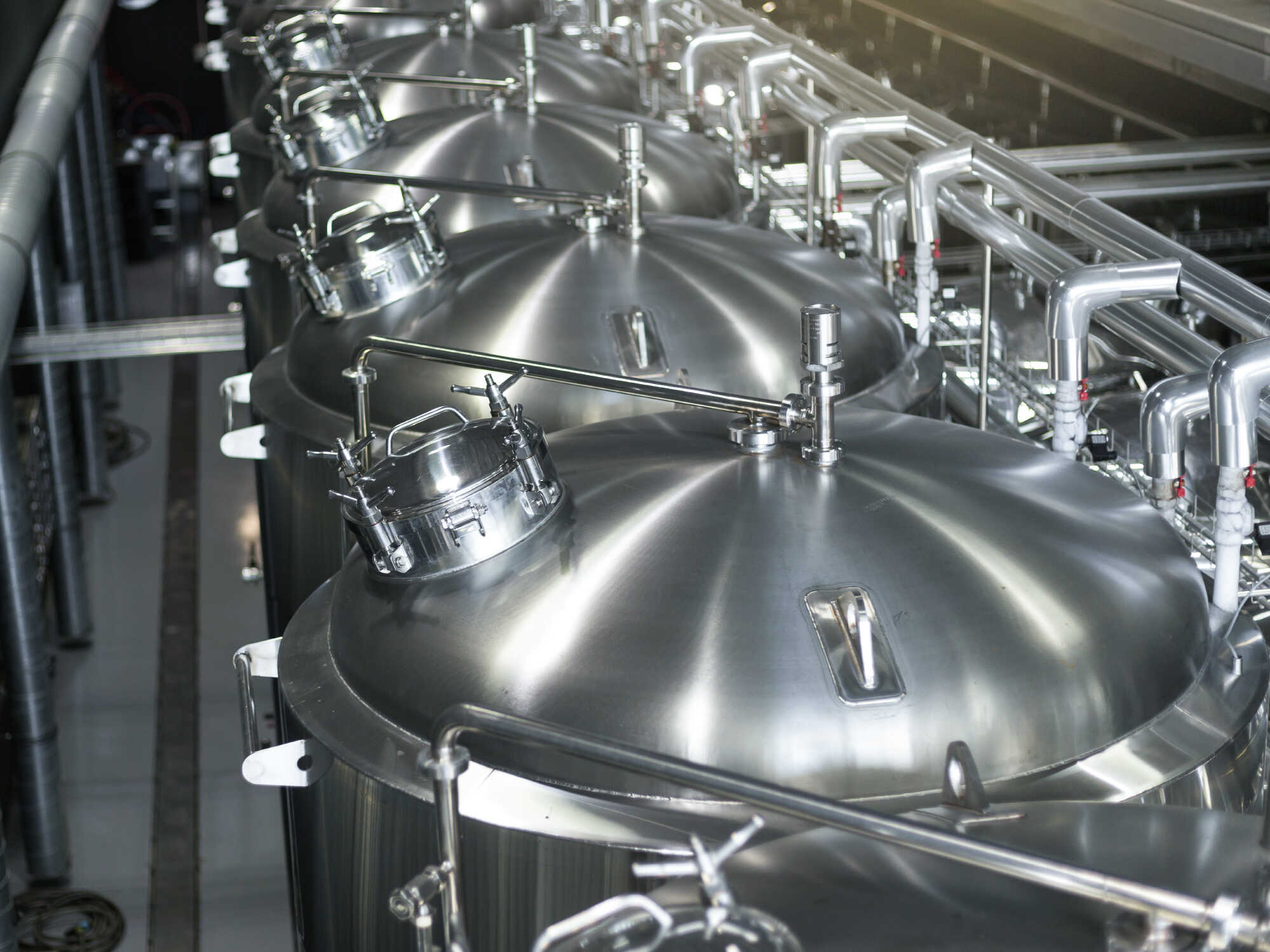
Deep dive: Fermentation upstream bioprocess design
Explore the processes, equipment, and bioreactors critical for commercial fermentation-derived ingredient production.
Overview of commercial fermentation bioprocesses
Fermentation biotechnology applies engineered biological processes (bioprocesses) using cells, enzymes, or cell components to produce a bioproduct from the metabolism of raw material feedstocks such as carbon and nitrogen. Biomanufacturing already produces chemicals, enzymes, food ingredients, materials, and pharmaceuticals from microbes. Using lessons from the bioindustrial and biopharmaceutical industries, advances in fermentation bioprocess design are enabling the production of food ingredients that can replicate animal-derived ingredients.
Fermentation is a bioprocess that uses microorganisms such as yeast, bacteria, and fungi to convert complex or simple carbon sources into compounds of interest (such as enzymes, metabolites, proteins, vitamins, or oils). Traditionally, fermentation is the microbial conversion of sugar into an organic acid or an alcohol. Food and beverage fermentation conventionally uses microbes to preserve and improve the organoleptic properties of foods like bread, beer, vinegar, yogurt, cheese, and wine (Paulová, Patáková, and Brányik 2013). Now the food industry is developing bioprocesses to create alternative proteins, fats, and other ingredients from microbes through more advanced fermentation processes. Here we describe fermentation in this new context as biomanufacturing bioproducts, including food ingredients, from different microbes and feedstocks beyond classical sugar fermentation to acids and alcohols.
To produce ingredients such as protein-rich mycoprotein biomass or precision fermentation-derived protein, the selected strains and feedstocks are mixed in a bioreactor. Bioreactors, sometimes called fermenters, are where specific biological processes convert feedstocks under controlled conditions. In addition to the essential bioreactor, a commercial fermentation process includes an array of equipment and associated steps from upstream to downstream production (Figure 1). Upstream processes (USP) include all the processing and preparation prior to harvest of the cells, such as substrate/medium preparation, medium sterilization, microorganism growth for inoculation (seed train), equipment sterilization, and finally, growth/production in the main bioreactor. Downstream processes (DSP) include all processing after cell harvest, such as dewatering, extraction, and purification of the fermentation product from the biomass (solid fraction) or supernatant (liquid fraction), along with equipment sterilization and wastewater effluent treatment (Gutiérrez-Correa and Villena 2010; Warner 2019).
Both USP and DSP are crucial to the success and economic viability of the fermentation bioprocess. However, because of the vast options and information in both areas, this deep dive will focus on the typical systems, strategies, and commercial advances in fermentation technology for USP development. A separate deep dive into DSP is warranted as these technologies can often be more complicated and costlier than the fermentation process alone.
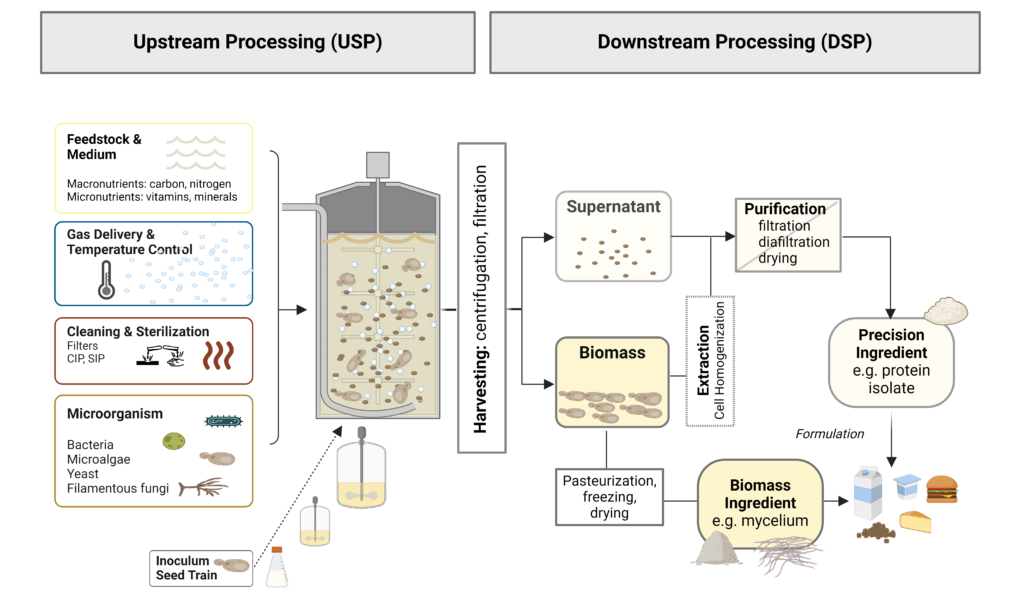
Bioreactor basics
In USP, microbes and sterile feedstocks are brought together in a controlled bioreactor environment to produce nutrient-rich biomass or a specific metabolite, including enzymes, proteins, oils, or other molecules. Bioreactors can be generally broken into submerged fermentation (liquid state) and solid-state fermentation (SSF) based on the substrate and continuous phase in which the microbe grows. Bioreactor sizes can vary from small benchtop systems (1-20L) at the laboratory scale to large-scale commercial bioreactors (50-1000 m3) for biomanufacturing. Commercial-scale bioreactor volumes are often reported in cubic meters (m3), where 1 m3 equals 1,000 L. Regardless of the scale and type of system, the main function of a bioreactor remains the same: to provide an optimal and controlled environment for the microorganism to produce the product of interest.
The typical inputs into the bioreactor include the media, nutrients, gasses, and the microbe. The main parts of a bioreactor depend on the design and application (submerged vs. SSF), but generally include a vessel, mixing system, inlet/outlet ports (for media, nutrients, inoculum), sterilization system, gas input, gas exhaust, temperature control, and various control systems. Process monitoring and control in a bioreactor involve various sensors and control strategies to optimize the conditions for cell growth, product formation, and yield. Monitoring typically involves measuring critical process parameters such as temperature, gas concentration (primarily dissolved oxygen), mixing rate, pH, humidity (most relevant for SSF), and substrate concentration in real time. This information is then fed into a control system that adjusts process parameters to maintain the desired conditions for optimal microbial growth and production (Gutiérrez-Correa and Villena 2010).
Any heat generated in the process must be removed from the system to maintain ideal growth temperatures for the microorganism. Temperature control is crucial to bioreactor operation as the temperature can greatly affect the growth rate, metabolism, and product formation. The temperature control system typically consists of a temperature sensor, a heating and cooling system, and a controller (H. J. Noorman et al. 2018). The heating and cooling system generally includes an integrated jacket, internal coils, or an external heat exchanger where the circulated water is cooled by a chiller or heated by a steam heat exchanger. The temperature sensor measures the current temperature inside the bioreactor, and the heating and cooling system adjusts the temperature as needed to maintain the desired set point (H. J. Noorman et al. 2018).
Gas delivery and control in a bioreactor provide key substrate gasses and ensure optimal gas mixing and heat transfer necessary for cell growth and production. Gas delivery, such as air, oxygen, and carbon dioxide, is regulated by airflow controllers like mass flow controllers or blowers to ensure flow rate control. The gas delivery system mixes the gasses in the desired proportions and introduces the air to the vessel. In submerged liquid fermentation processes, gas is delivered through a sparger, a submerged air diffuser found at the bottom of the vessel. Gas flow is typically expressed as air delivery volume per volume of liquid per minute (VVM) to standardize the gas flow rate across different vessel sizes (Zhang et al. 2019). In SSF, gas ventilation serves the same function in gas exchange and can be delivered through gas diffusers and blowers. Air ventilation in SSF also has a strong role in providing adequate heat exchange in the bioreactor (Ge, Vasco-Correa, and Li 2017). For both submerged and SSF, gas control is often monitored through in-line sensors like dissolved oxygen probes and exhaust gas monitors.
Each fermentation system type has different bioreactor components and controls based on the system needs. For instance, submerged fermentation systems must maintain controlled pH ranges for the microbe through inline probes and acid or base addition. For SSF systems, humidity monitoring and control are more highly prioritized considerations.
Types of upstream fermentation technologies
Submerged and solid-state fermentation
Many different bioreactors can be classified in several ways, such as by gas transfer, agitation type, water content, feedstock, and temperature. In general, bioreactors can be classified as either submerged or SSF systems. In submerged fermentation, the substrate to be fermented is always in a liquid phase along with the nutrients required for microorganism growth, and gas exchange occurs via mixing. By contrast, in SSF, the microorganism grows on a predominantly solid substrate with gas delivery through aeration and a reduced liquid phase (Sun, Shahrajabian, and Lin 2022). There can be technology overlaps between the two fermentation systems, though, such as with liquid-air interface fermentation. See Table 1 for key features and differences in submerged and SSF systems.
Submerged fermentation, the most common industrial fermentation process, enables a tightly controlled environment for optimal production with diverse microorganisms and feedstocks (Table 1). (Paulová, Patáková, and Brányik 2013). This includes bacteria, microalgae, yeast, and other fungi across many metabolism types.
Beer production is an example of a traditional submerged fermentation process in which fermented sugars are converted to alcohol under anaerobic and often unmixed conditions. The majority of precision fermentation products, like enzymes, are produced through submerged fermentation because of the tight, reproducible control needed over the system. However, the energy costs, water usage, and feedstock costs can be much higher in submerged than in SSF processes.
Submerged | Solid-state |
---|---|
Water is main component of culture | Reduced or no free-flowing water |
2-phase system: liquid – gas Liquid is the primary phase for temperature control and gas exchange. Gas is delivered through the liquid phase for mixing and gas transfer. | 3-phase system: solid – gas – liquid Gas is the primary phase for temperature control and gas exchange. Gas is delivered across solid and/or liquid interfaces for heat and gas transfer. |
Homogenous system; good mixing | Heterogeneous system; poor mixing |
Dissolved nutrients in water; lower average concentration of nutrients | Complex insoluble substrate; higher average concentration of nutrients in feedstock |
High feedstock material cost (usually); Able to utilize gas feedstocks | Lower feedstock material cost; Able to utilize different agricultural sidestreams |
Applicable to a wide range of microorganisms | Limited range of microorganisms used |
Microorganisms grown in solution; Absorb nutrients from the liquid medium; Little to no nutrient gradient when mixed. | Microorganisms adsorb to or penetrate substrate surface (typically); Absorb nutrients from the solid substrate; Nutrient gradient exists |
High energy usage | Lower energy usage |
Good process control and monitoring (e.g., temperature, pH, DO, nutrients) | Reduced and poor process control and monitoring |
Better heat and mass transfer | Limited heat, nutrient, and gas transfer |
Final harvest is a liquid with lower product concentrations | Final harvest is a wet substrate with high product concentrations |
Robust DSP options | Difficult to separate substrate from the final product |
The other type of bioreactor fermentation used in the food industry is solid-state fermentation (SSF), also called solid substrate fermentation. SSF is a fermentation process where the microorganisms are cultivated on moist, solid, non-soluble organic material with little to no free water. The solid substrate, such as grains, seeds, legumes, husks, peels, or other organic materials, acts as a support and nutrient source for microorganism growth (Yafetto 2022). SSF originated in traditional food fermentation to produce foods like bread, tempeh, miso, and koji. For example, tempeh results from steamed soybean fermentation by the fungus Rhizopus, while koji is the product of cooked rice fermentation by the fungus Aspergillus.
The industry has seen an increased interest in SSF for producing many bioproducts, including enzymes, organic acids, mushrooms, single-cell proteins, secondary metabolites, and more. The microorganisms used are typically fungi, which are suited for SSF because their hyphae can grow into the solid substrate, penetrating the inter-particle space and digesting cellulosic material (Yafetto 2022). SSF can be a valuable food processing approach for bioconverting organic agro-industrial wastes like husks, straw, and peels, which cannot be as readily implemented in submerged fermentation, into bioproducts. Fungi are predominantly used in SSF to produce enzymes, citric acid, fermented animal feed, single-cell protein, and mycoprotein. Only some yeast and bacteria are used in solid-state fermentation to produce organic acids like acetic acid (Sun, Shahrajabian, and Lin 2022). In the alternative protein space, SSF holds great promise for creating whole-cut meat alternatives and improving plant-based feedstocks’ organoleptic and nutritional properties. There is untapped potential for SSF use in precision fermentation to reduce commercial costs.
The primary advantages of SSF are the simpler design, lower capital cost, reduced contamination risk, lower water usage, lower energy usage, and the usage of underutilized feedstocks. However, SSF is limited by substrate heterogeneity, slower organism growth, feedstock sterilization, and limited gas and heat transfer rate (Table 1). In addition, imprecise process control over parameters like temperature, pH, dissolved oxygen, and nutrient availability is a key limitation of SSF (Paulová, Patáková, and Brányik 2013). SSF is also partially limited by the types of microorganisms that thrive in low-moisture environments (Sun, Shahrajabian, and Lin 2022).
It is difficult to cover the diverse bioreactors used for microbial fermentation comprehensively. Below are the typical bioreactor types used in the alternative protein sector, though, there is always room for new bioreactor designs or the application of other bioreactors to the alt protein sector.
Submerged fermentation bioreactors
A typical submerged fermentation bioreactor is an upright, closed, cylindrical stainless steel tank, such as a stirred tank bioreactor (STR) or an airlift bioreactor. The basic features typically include a headspace volume, agitation system, gas delivery system, gas exhaust port, temperature control, acid/base inlet, sampling port(s), cleaning fluid port, drain port, medium, and nutrient delivery ports, and aseptic inoculum port (Gutiérrez-Correa and Villena 2010). All submerged fermentation bioreactor design is dictated by liquid mixing, gas mass transfer, and heat transfer.
Mixing affects cell homogeneity, heat transfer, and cell access to feedstock, nutrients, and oxygen. The mixing and gas delivery impacts mass transfer of gasses from the bubbles to the cells via the liquid phase (H. J. Noorman et al. 2018). Gasses are delivered via spargers in most reactors, but the mixing and distribution depend on the bioreactor design. Temperature control in submerged fermentation reactors generally includes either an integrated jacket, internal coils, or an external heat exchanger. Cooling is accomplished by chiller water heat exchangers or via the integrated jacket with circulating chiller water. Steam heat exchangers typically provide heating. The method for cooling and heating depends on the bioreactor type and scale. Process control in a submerged fermentation reactor is tightly controlled through mixing, temperature exchange, gas exchange, nutrient dosing, and pH regulation (Gutiérrez-Correa and Villena 2010).
Most commercial submerged processes are aerobic, with the microorganism requiring oxygen (i.e., aerobic heterotrophs). Thus, adequate gas-liquid exchange is required for growth. For aerobic fermentation, the extent of gas-liquid exchange is characterized by the oxygen transfer rate (OTR; mmol L-1 hr-1) and the volumetric mass transfer coefficient (kLa), which details how efficiently oxygen is transferred from the gas phase to the liquid medium. Gas mass transfer is greatly affected by pressure, temperature, vessel geometry, medium viscosity, gas bubble size, gas delivery rate, and agitation or mixing (Gutiérrez-Correa and Villena 2010). For instance, smaller bubbles increase the surface area for gas diffusion, and higher temperatures reduce gas solubility, while higher pressures increase gas solubility (Henry’s Law). For other microorganisms, gas exchange of feedstocks like methane, hydrogen, carbon monoxide, carbon dioxide, and other gasses is critical for growth. For example, industrial processes with methanotrophs, hydrogen oxidizing bacteria, and phototrophic microalgae rely on gas feedstock fermentation. The mass transfer rate of any gas feedstock is an important control parameter and a primary design consideration in submerged fermentation bioreactors.
Below are the typical submerged fermentation vessels used commercially (Figure 2):
Stirred-tank bioreactor (STR)
A stirred tank reactor (STR) is the most common type of submerged bioreactor. These reactors are closed cylindrical tanks equipped with mechanical agitation systems consisting of an external motor drive, agitator shaft, impeller for radial or axial flow, and baffles on the tank sides to increase fluid turbulence and prevent a vortex (H. J. Noorman et al. 2018). Gasses are delivered through a perforated sparger at the bottom of the tank. Stirred agitation allows for improved gas dissolution and distribution along with microorganism and nutrient distribution throughout the bioreactor to ensure a uniform environment (Gutiérrez-Correa and Villena 2010). STRs offer good mixing for high viscosity medium due to the agitator shear mixing, which can be beneficial for high productivity precision fermentations with yeast, for example. However, the high mixing can cause foaming, which can negatively impact gas venting and equipment. Moreover, high shear may not be desirable if the microbe is shear-sensitive or if a specific cell morphology must be maintained, such as with fungal mycelial or filamentous biomass. STRs are often used at laboratory-to-pilot scale from 0.25-100L for research purposes, process optimization, or small-scale production. Commercially, STRs have widespread usage with volumes up to 150-200 m3 but are generally more expensive to build and operate than smaller systems. For example, in even larger STR (such as 500 m3), sufficient mixing requires an immense energy input increase, which often makes it economically unviable compared to bubble columns and airlift reactors (Warner 2019, H. J. Noorman et al. 2018).
Bubble column bioreactor (BC)
Bubble column (BC) reactors are attractive for their simple, cost-effective design and lower energy costs. In a BC reactor, gas is sparged through a column containing the liquid medium and microorganisms, and mixing takes place due to the upward movement of gasses through this column (Gutiérrez-Correa and Villena 2010). The sparger nozzle at the bottom of the reactor must be evenly distributed to ensure homogenous flow and mixing. Sieve plates or columns can be added to facilitate mixing and dispersion (Gutiérrez-Correa and Villena 2010). A disadvantage of BC reactors is reduced liquid circulation between upper and lower portions, which can be overcome by a recirculating pump or through an airlift bioreactor. BC reactors can be run up to a 1000 m3 capacity, such as in citric acid production with Aspergillus niger (Paulová, Patáková, and Brányik 2013). The production of bakers’ yeast (S. cerevisiae) is one of the oldest and most common bioprocesses and usually takes place in large aerobic BC fermenters (100-300 m3) using molasses as a feedstock in a fed-batch process (H. J. Noorman et al. 2018).
Air-lift bioreactor (ALR)
An air-lift bioreactor (ALR) is a modified version of a BC reactor with a separated loop whereby gas drives the liquid culture up a riser, and then the liquid culture falls back down through a downcomer, improving liquid mixing over a BC reactor. The loop can be internal to the system using baffles or barriers or an external loop where the degassed liquid returns through a separate downcomer. The pressure difference between the riser and the downcomer aids in fluid circulation (Merchuk and Gluz 2002). There are many variations of the ALR using the loop principal to mix the bioreactor. For example, Quorn uses an external loop ALR driven by an airlift riser to grow its Fusarium venenatum mycoprotein (Moore, Robson, and Trinci 2019). ALRs are often used at large-scale volumes, such as Quorn’s 150 m3 ALR, as they allow for well-mixed gasses, reduced cell shear, lower power consumption, and reduced capital cost.
Gas or loop bioreactor
U-loop bioreactors are gas-liquid contactor vessels often used in gas fermentation and are similar to external ALRs in terms of liquid flow path. However, gas is not the driving mixing force in the U-loop bioreactor. A pump system facilitates mixing to circulate the liquid. The gas is introduced at the top of the reactor downcomer tube or introduced elsewhere along the flow path, where the liquid phase is recirculated downward faster than the gas bubble rise rate. This facilitates very efficient gas-liquid mass transfer (Olsen et al. 2020). These reactors are used in gas fermentation with methane and other gaseous carbon sources to produce single-cell proteins and biofuels. Commercial examples include Unibio and Calysta, producing single-cell protein through methanotrophic gas fermentation.
Photobioreactor (PBR)
A photobioreactor (PBR) is used to cultivate photosynthetic microalgae and cyanobacteria, which utilize light as an energy source and carbon dioxide as a carbon source. The general design optimizes cost, light utilization, and carbon dioxide delivery (gas mass transfer). PBRs can take many forms as either a closed system or an open system. Open photobioreactors are typically raceway ponds, which are mixed by a paddle wheel, open to the environment, and relatively inexpensive to build and operate (Gutiérrez-Correa and Villena 2010). Closed photobioreactors are characterized by a transparent tubular vessel or panel array that is optimized for light delivery outdoors or when outfitted with a light source. Closed systems can include modified BCs, airlift PBRs, tubular plug flow reactors, and more. These systems provide more control, higher productivity, and contamination prevention but often come at a higher capital and operation cost. Thus, PBR systems often produce high-value, low-volume products like nutraceuticals, cosmetics, and pharmaceuticals (Araújo et al. 2021). In food, PBR systems are utilized for the production of Arthrospira (Spirulina) biomass for its nutrition and ease of production, while the carotenoid astaxanthin from Haematococcus has been an attractive antioxidant ingredient due to its high functionality and market value at over $1,000 per kg (Stachowiak and Szulc 2021).
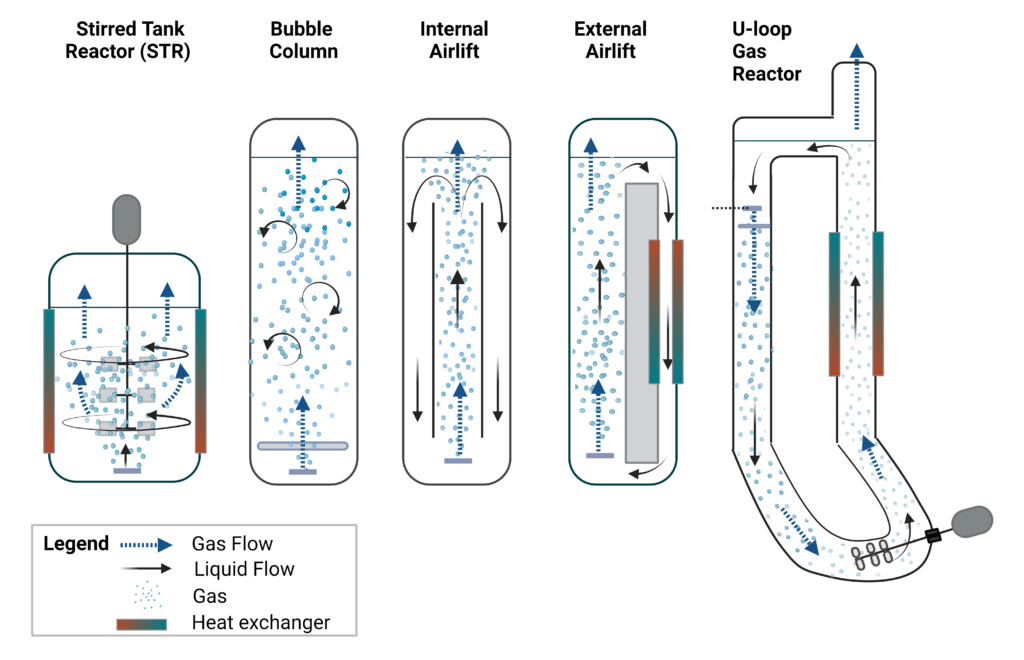
Solid-state fermentation bioreactors
The many advantages of solid-state fermentation (SSF) suggest it has abundant economic potential. However, its industrial applications are not as diverse as for submerged fermentation, and a key limitation is a lack of simple and practical automated fermenters for SSF processes. Since the predominant phases in SSF are gas and solid, the system is not as homogeneous for monitoring and control, with the potential for poor mixing and heat removal (Table 1) (Chen 2013). Successful SSF application relies heavily on bioreactor design and precise operation due to the heterogeneous mixture and poor heat and gas mass transfer.
Traditionally, SSF bioreactors can be classified into static and dynamic systems based on their mixing. These can also be classified as stationary, intermittent, or continuous stirred processes with ventilation as either natural convection or forced air (Chen 2013). Both agitation and ventilation have a strong impact on temperature control and mass transfer. Gas ventilation is a primary mechanism to drive temperature and humidity or moisture control in SSF bioreactor systems, though some SSF reactors use jacketed cooling systems for heat removal (Chen 2013).
The four predominant types of SSF bioreactors (Figure 3) are (1) reactors without mixing and forced aeration (tray reactors), (2) reactors without mixing but with forced aeration (packed-bed bioreactors), (3) reactors with mixing but no forced aeration (stirred drum bioreactors), and (4) reactors with mixing and forced aeration (stirred-aerated bioreactor) (Ge, Vasco-Correa, and Li 2017).
Tray bioreactor
Using trays is the most common and oldest system due to their very simple design. Tray reactors are used in static conditions with no mechanical agitation — these include traditionally unmixed beds with no forced aeration on the solid substrate (Manan and Webb 2017). Tray bioreactors consist of a chamber containing stacked trays, where the air is circulated around the trays to facilitate gas exchange and temperature and humidity control (Couto and Sanromán 2006). Trays can be outfitted with perforated mesh bottoms to facilitate airflow through the substrate or as a solid tray where the air is only ventilated over the tray top. Heat exchange and gas mass transfer through the beds rely only on diffusion since there is no mixing and no forced air blown through the substrate beds. Thus, the tray only holds a limited amount of solid substrate to facilitate exchange. It is a simple design that has been traditionally used in tempeh and koji production (Ge, Vasco-Correa, and Li 2017). This design can be unattractive at large scale due to the number of trays and operations needed, though automation can help. Nature’s Fynd has modified the tray bioreactor to be cultivated with a liquid substrate instead of a solid, which they call liquid-air interface fermentation. This is discussed in the commercial case studies section.
Packed bed bioreactor
A packed bed bioreactor is a tubular container with the solid substrate and microorganism packed in a column with a perforated base that provides support and ventilation. These reactors are not mechanically mixed but have forced aeration through the bed either from the top or bottom, which facilitates gas mass transfer through the substrate and aids in heat transfer. The forced air is saturated with water for humidity control and prevents the substrate bed from drying out. The tubular, packed design also enables the use of a water jacket for cooling and heating, which enables better temperature control (Ge, Vasco-Correa, and Li 2017). However, heat transfer is still dependent on the width of the bioreactor due to a lack of mixing. As the microorganisms grow, especially fungal hyphae, and consume the packed substrate, channeling in the bed can form, which creates agglomerates, undesirable airflow, and areas of reduced ventilation. Thus, a drawback of this system is non-uniform growth and difficulties harvesting the product (Couto and Sanromán 2006). Despite this drawback, the traditionally packed bed bioreactor is commonly used in rice koji production and extensively studied for enzyme production, such as pectinase produced from Aspergillus niger.
Rotating/stirred-drum bioreactors
As the name implies, the stirred-drum bioreactor is a cylindrical, horizontal drum partially filled with substrate and microorganisms. Mixing is accomplished by either paddle/stir mixers or drum rotation on its central axis (Ge, Vasco-Correa, and Li 2017). Ventilation is supplied by air blown through the headspace. There is no direct ventilation of the substrate bed, but the mixing facilitates improved gas mass transfer and heat exchange. Temperature can be further controlled by a water jacket in the drum. Another variation of the stirred-drum bioreactor is a plug-flow bioreactor (PFBR), a horizontal reactor that moves substrate axially through the reactor using an auger while gas ventilation can flow above the surface. PFBR offers an opportunity for semicontinuous operation in SSF.
Stirred-aerated bioreactors
These bioreactors have mechanical mixing and forced aeration to promote better substrate homogeneity, heat transfer, and gas mass transfer. Examples include fluidized bed reactors, stirred-aerated bed reactors, and rocking-drum reactors. Fluidized-bed bioreactors are similar to packed-bed bioreactors; however, the bed is forcefully aerated to fluidize the particles and mix the system (Ge, Vasco-Correa, and Li 2017). Like a stirred-tank bioreactor, it can be outfitted with an impeller at the bottom to break up agglomerates and further fluidize the bed. Comparably, the stirred-aerated bed reactor has a semi-packed bed that is aerated from the bottom and mixed by a vertical mechanical agitator inside the unit. Lastly, the rocking drum reactor is a modification of the stirred-drum bioreactor, which consists of three drums: inner, middle, and outer. The substrate is loosely packed in the middle drum, which is perforated for airflow between the inner, middle, and outer drums. Air is delivered through the inner drum, facilitating gas ventilation through the middle substrate and into the outer ‘headspace’ drum (Ge, Vasco-Correa, and Li 2017). All three stirred-aerated bioreactors can have a water jacket for temperature control.
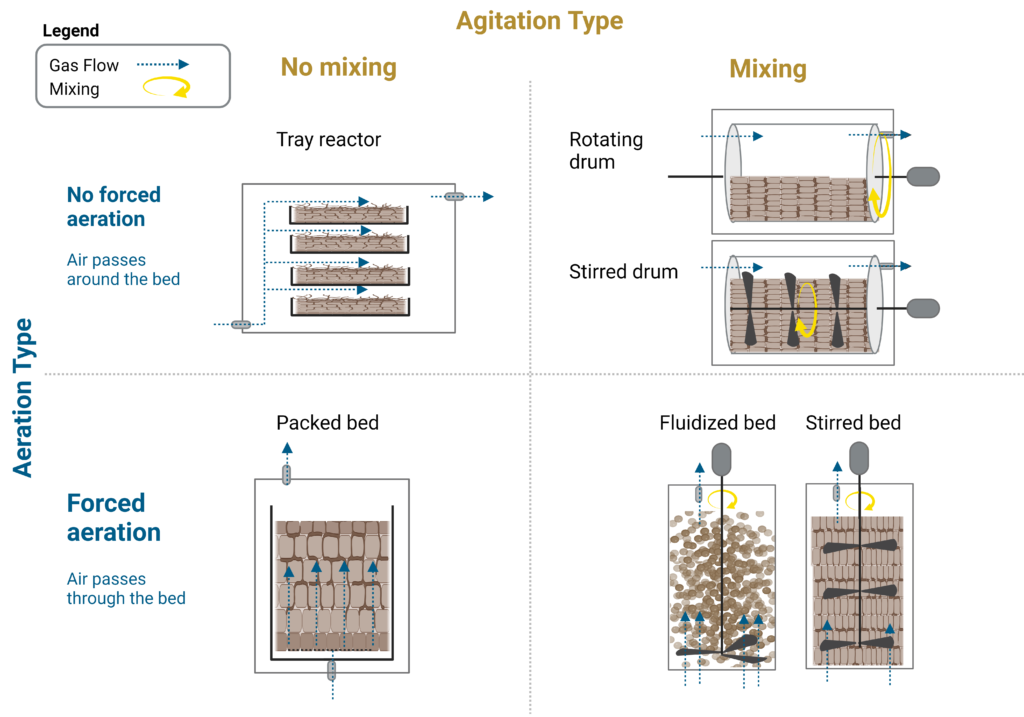
Upstream bioprocesses
A primer
In a commercial USP, one must prepare the medium, prepare the inoculum culture, sterilize the bioreactor and equipment, combine the seed culture and sterile medium, and then perform the production process. The fermentation process must then be monitored and controlled for optimal productivity and then harvested for the DSP.
The bioreactor, associated equipment, and all inputs, including gas and medium, should be cleaned and, in some cases, sterilized before use. The aim is to remove or reduce any contaminating microorganisms that may impact the productivity and quality of the production run. After a production run and before any new medium or organisms enter the system, all bioreactor systems (including piping and tanks) should be cleaned in place (CIP) using alkaline and/or acid treatment. A typical CIP process includes rinsing, a hot caustic soak with a base such as NaOH, a reverse osmosis (RO) water rinse to neutralize the base, an acid soak to descale and sterilize, and a final RO water rinse to neutralize (Chisti and Moo-Young 1994). Commercially, this requires CIP-fluid holding tanks and dedicated metering pumps for precise chemical delivery to the CIP water streams. Figure 4 depicts a generalized commercial upstream fermentation bioprocess system, including the CIP equipment.
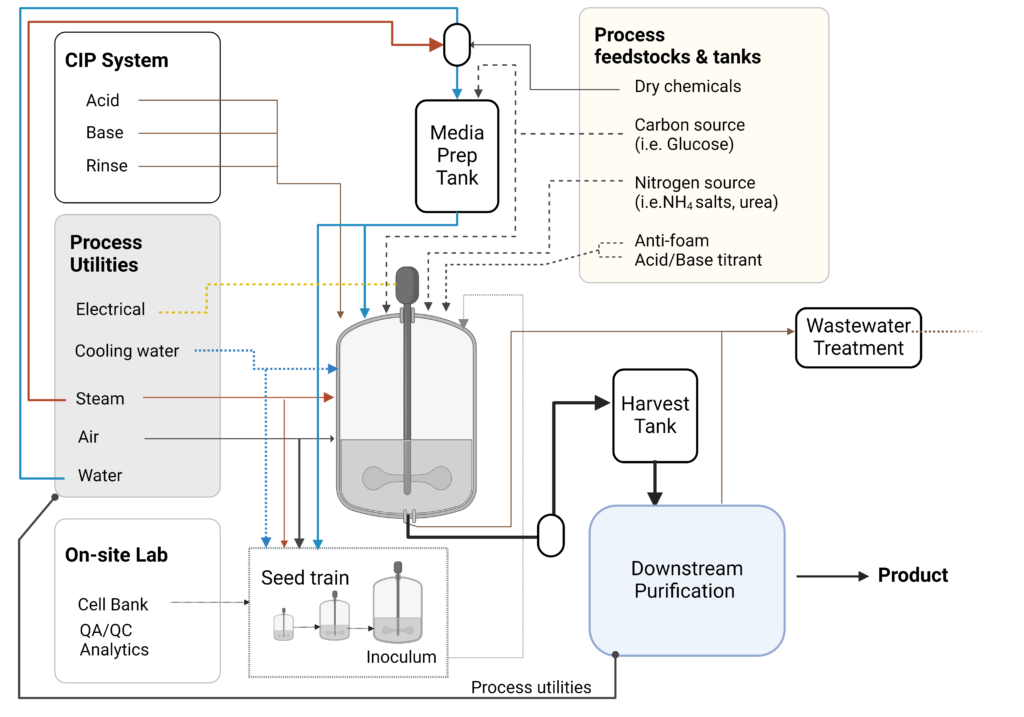
Substrate and cultivation medium preparation
The substrate and cultivation medium must be prepared before fermentation. This usually occurs in a separate medium preparation tank to combine all components. Before and during the fermentation process, the gas, medium, and nutrient inputs should also be sterilized. Sterilizing the gas and medium inputs includes chemical, thermal, radiation, and filtration or other physical removal processes(Gutiérrez-Correa and Villena 2010). In the lab, medium and nutrient sterilization usually consists of simple filtration and/or autoclave high-temperature sterilization. Commercially, medium and nutrient sterilization can take several forms as either batch sterilization or continuous sterilization (Figure 5).
Batch sterilization in place (SIP) is often performed in jacketed bioreactors where the medium and vessel are pressurized and heated to at least 120°C using steam input through the jacket and then rapidly cooled using water from a chiller (Figure 5a). In continuous sterilization, the components can be sterilized through an in-line filter or through a high-temperature short-time (HTST) treatment (Figure 5b). A HTST continuous sterilization can improve steam efficiency and reduce thermal damage to the medium with efficient cell disruption of unwanted cells, which is helpful if liquid medium components are heat sensitive or needed on a continual, on-demand basis during the production run (Cabansis et al. 2016). For instance, HTST sterilization can deliver nitrogen hydrolysate (peptones) and sugar feedstock (such as liquid glucose, Dextrose Equivalent 95) independently to prevent Maillard reactions that can occur when dosed together. These HTST sterilizers also enable dosing throughout the production run. These sterilizers are run at different temperature setpoints depending on the medium components and are often used in downstream sterilization or pasteurization processes as well as USP.
If a solid substrate is used, such as in SSF, the material can be autoclave sterilized at lab and pilot scale. For commercial bioprocesses, solid substrates can be sterilized through heat treatment such as steam explosion, pasteurization, or even irradiation or chemical pasteurization processes that can be conducted on a conveyor system or large format autoclaves can be used, depending on the production scale.
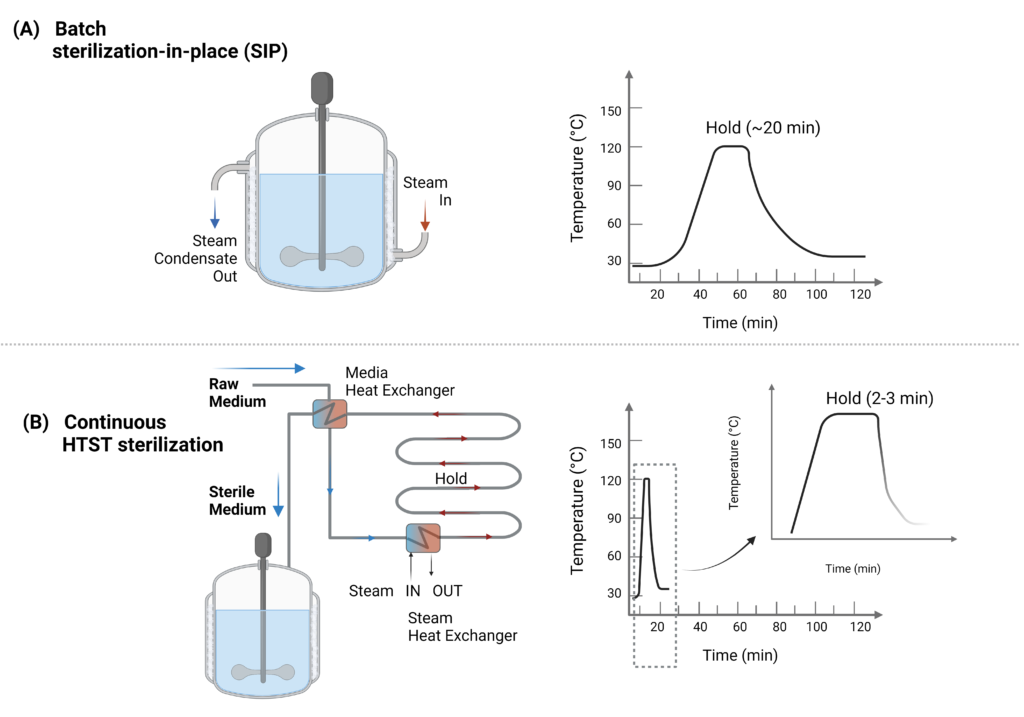
Seed train inoculation
To inoculate the production bioreactor, the microorganism must be scaled up through a series of seed fermenters called the seed train. This seed train often originates from a quality-controlled, cryopreserved working cell bank. This working cell bank is then scaled up from a small scale (such as a plate or flask) to sequentially larger fermenters until the appropriate cell density and volumes are obtained for inoculation into the production bioreactor. Often, each expansion step is performed at a 10x volumetric expansion (e.g. 5L to 50L, to 500L, etc.). For commercial operations, this can add significant time and capital depending on the organism and type of fermentation system.
Once the microbial seed culture and sterile medium are combined in the bioreactor, the production run begins, during which the temperature, pH, dissolved gasses, and nutrient levels are maintained at desired levels. Optimizing growth and controlling these parameters will largely depend on the type of microorganism, cultivation mode, and bioreactor system.
Productivity & optimization
Microorganism growth and productivity in a bioprocess
The upstream fermentation goal is to grow the microorganisms to a suitable and productive biomass density with sufficient cells or cell-derived ingredients for harvest. For biomass products, more cells equal more product. For precision fermentation, more cells equal higher biological production of the target molecule, such as a protein, lipid, or other biomolecule. Understanding the growth kinetics of the microbe is crucial for large-scale bioprocess so that the inoculum, feedstocks, and conditions can be optimized for productivity.
When grown in a closed system with limited nutrients, the growth of any microorganism can be broken into four phases: (1) inoculation or lag phase, (2) exponential growth log phase, (3) stationary phase, and (4) decline or death phase (Figure 6). At the beginning of cultivation, viable cells from the seed train are inoculated into the bioreactor filled with a sterilized medium. The lag phase occurs after inoculation with slow growth as cells adapt to a new environment. Once adapted, cells grow proportionally to cell mass exponentially until nutrients or other limiting factors slow the growth during the deceleration phase. Growth can be slowed due to many factors, such as nutrient or gas transfer limitation, toxic compound accumulation, or product inhibition. Once slowed, cells enter a stationary phase where cell division and cell death reach equilibrium. Finally, cells reach the decline phase, where the rate of cell death is greater than cell generation (Gutiérrez-Correa and Villena 2010). In a typical process, the late log phase and early stationary phase are the most productive due to the high number of healthy cells.
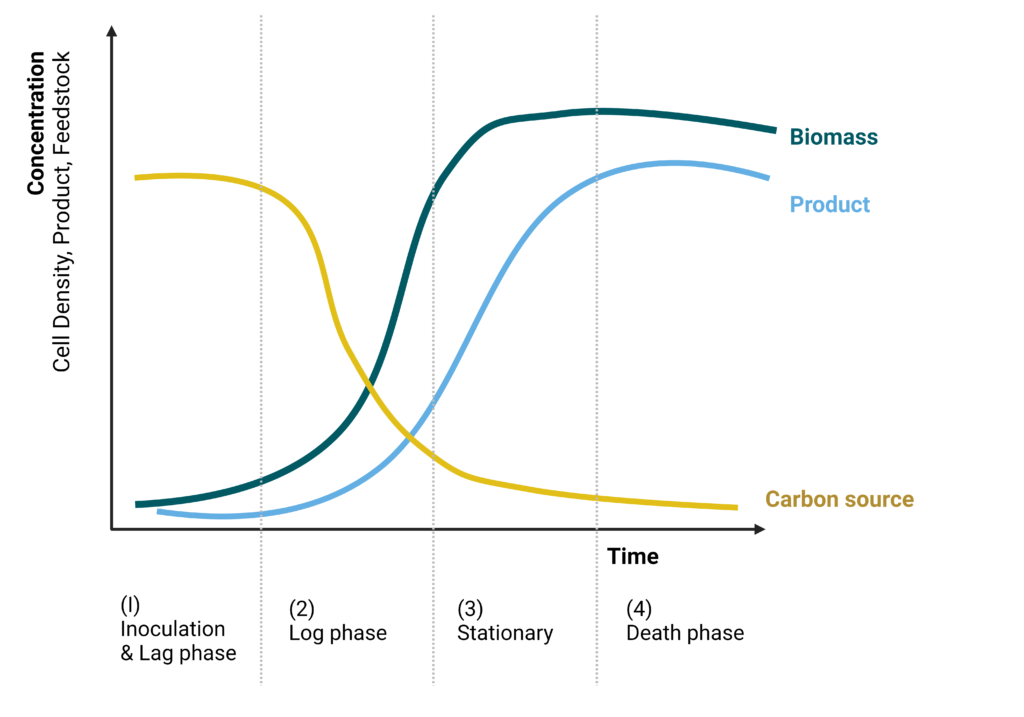
For biomass or precision fermentation, commercial productivity metrics can be broken into biomass/product titer, rate, and yield (TRY).
Titer
Titer is the mass of the expressed product relative to the total upstream liquid bioreactor volume containing the product in submerged fermentation. In SSF, the titer is expressed as the mass of the product relative to the mass of the solid substrate used or concentration in extracted liquid. The titer is typically reported in terms of gram per liter for submerged processes or gram per gram substrate in SSF. A higher product titer can greatly reduce downstream processing costs and time due to the higher initial product content due to the need to concentrate and dry the products, whether biomass, protein, or lipids. A higher titer can require less product concentration or water removal, improving energy usage and preventing product losses during DSP, which can also reduce equipment sizing requirements and possibly DSP operation steps.
Rate (productivity)
Productivity rate is a temporal measure of production titer, which is expressed as the amount of biomass or target product produced relative to the total upstream liquid volume over time (i.e. g-biomass/L/day; g-protein/L/day). In an SSF process, the productivity can be measured against the total substrate mass (SFF) over time (i.e. g-biomass/g-substrate/day). Higher productivity can equate to shorter batch durations, which improves annual output productivity due to faster production and increased batch numbers.
Yield
Biomass or precision fermentation yield measures how much product is produced per unit of feedstock added to the system, which is typically expressed in terms of grams per gram substrate (i.e. g-protein per g-glucose). Since the carbon feedstock (typically glucose) adds to operational costs, this acts as a measure of carbon use efficiency from the microorganism. Higher feedstock yields improve unit economics and sustainability of a process. Upstream feedstock yields are separate from downstream process (DSP) yields, which measures the amount of product recovered from start to finish after harvesting (i.e. g-product final per g-product initial).
Increasing the titer, productivity, and yield in a process significantly improves process OPEX and CAPEX. Thus, these values are the primary benchmarks for upstream efficiency in submerged and SSF bioprocesses. These are routinely monitored in submerged fermentation, however, it can be more difficult to track in SSF due to substrate and product heterogeneity.
Titer, productivity, and yield can be increased through technological advancements. These include improved expression systems, strain selection, strain development, medium optimization, feedstock delivery strategies, improved bioprocessing through automation or continuous cultivation, improved process analytical technology via improved sensors or machine learning, and through model-driven process optimization.
Cultivation operation modes
Improving bioprocesses through different cultivation modes is a hallmark of upstream bioprocess development. To achieve higher fermentation performance for either biomass or product titer, cultivations can be run in several operation modes, such as batch, fed-batch, continuous, and semi-continuous (Figure 7). Each operational mode has its advantages and disadvantages.
A typical fermentation run across all industries is a batch process. In a batch run, a single fixed volume of medium is provided for the microbial culture for each harvest (Figure 7a). As the microorganism grows and uses the nutrients, biomass and by-products are generated, and growth slows as it enters stationary growth as previously described (Paulová, Patáková, and Brányik 2013). Many industrial processes are run in batch mode due to the relative simplicity of the process. However, a batch can be limited by substrate availability and/or oxygen mass transfer during the exponential growth phase, which limits maximum productivity and yield.
A fed-batch process is similar to a batch. However, once the microorganism consumes one or more substrates in the medium, the additional substrate is aseptically added to the bioreactor while the product accumulates in the bioreactor (Figure 7b). This extends the active growth phase and productivity rate, which can be further tailored by adjusting the feed rate over time (Bolmanis et al. 2023). Feeding systems can be implemented for both the carbon substrate and/or nitrogen source used.
The advantages of fed-batch include a prolonged productivity period, the ability to achieve higher maximal cell density, the capacity to increase productivity through controlled nutrient additions, prolonged productive run time, and avoiding substrate inhibition at higher concentrations (Paulová, Patáková, and Brányik 2013). Another advantage of fed-batch operation is the ability to change substrates during a production run, which can be used to modulate metabolism and toggle gene expression for target pathways and products. This is often implemented in production using the methylotrophic yeast Komagaetella phaffii (previously Pichia pastoris) by initially growing on glycerol for high biomass production and then switching to methanol at high cell densities to turn on recombinant protein production via the AOX promoter (Nieto-Taype et al. 2020).
At the end of the batch growth phase, a prolonged steady-state phase equilibrium can be obtained through a continuous culture process. A continuous process includes the addition of fresh medium continuously to the bioreactor while a proportionate volume of culture broth containing cells, product, and spent medium is removed from the system. The harvest and feed rates are typically set to keep the cells proliferating at a fixed constant rate (Figure 7c) (Nieto-Taype et al. 2020). These can be operated as either (a) chemostat systems where a defined media addition and removal rate is used to control cell growth, or as (b) turbidostat systems where media addition and removal is controlled on a feedback loop based on the measured cell density (Paulová, Patáková, and Brányik 2013).
The benefit of a continuous mode is the possibility of long-term product synthesis, the ability to achieve stable product quality through continuous harvest, and a reduction in unprofitable downtime between production runs, all of which improve operating efficiency costs. However, the continuous process is operationally complex with constant DSP needs, which can increase contamination risk and create genetic drift over the course of long-term operation (Paulová, Patáková, and Brányik 2013; Li et al. 2014).
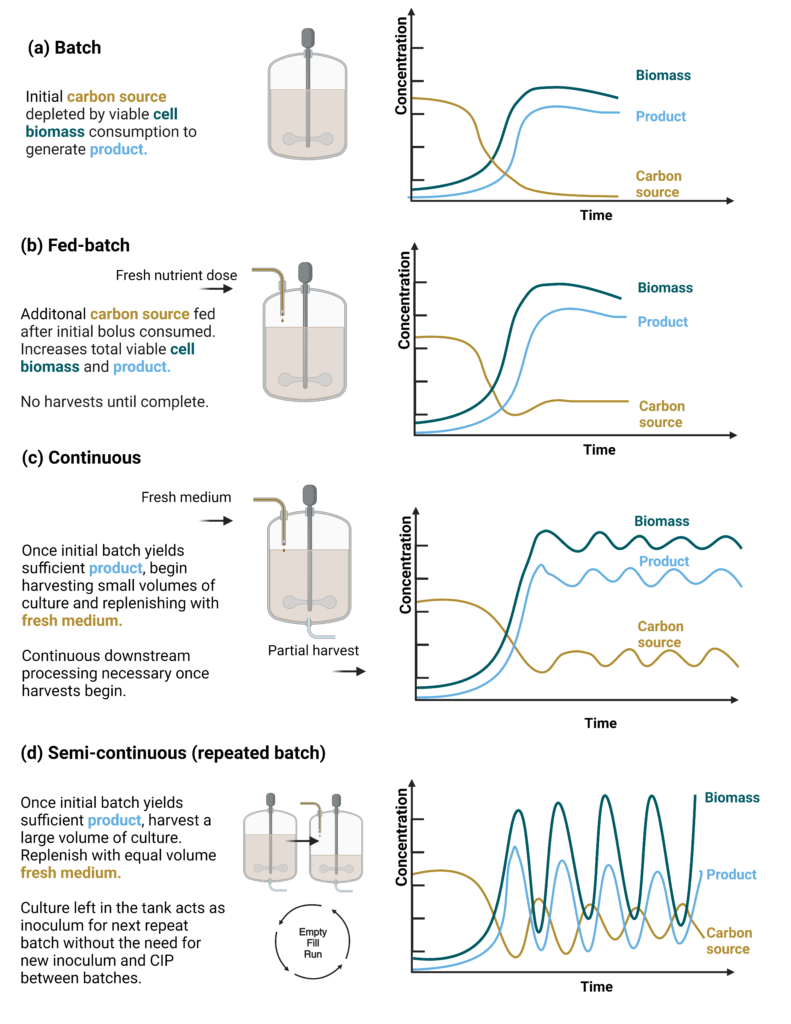
The final operation mode that is often considered is a semi-continuous or repeated fed-batch process where harvesting all but a small portion of the completed batch culture leaves the remaining cells for use as inoculum in the next filling cycle (Figure 7d). It is a simple concept but potentially difficult in practice. This mode allows for batched, higher-volume harvested material that can be processed in a single DSP run. This mode also increases operational ‘up-time’ by reducing seed train cycle numbers and CIP cycles between batches. However, a semi-continuous operation can also increase the operational costs and risk of contamination and genetic instability as the number of cycles progresses (Li et al. 2014).
Operation in fed-batch and (semi)continuous cycles mostly applies to submerged fermentation systems, which allow for the straightforward addition and removal of liquid media. Most SSF bioreactors are limited to batch mode operation due to the nature of the substrate and end product. For example, Nature’s Fynd produces its Fusarium mycelial mats through a batch tray reactor process. While most SSF is a batch process, some SSF bioreactors have been designed that allow for (semi)continuous operation. In fact, Nature’s Fynd has developed a now patented continuous or semi-continuous surface fermentation bioreactor system to produce their fungal protein, Fy. The process uses a roller to harvest the fungal biomats when mature, which provides increased automation and throughput over a more simplified tray-based air-liquid surface fermentation process. Other researchers have demonstrated semi-continuous SSF of stale bread by edible fungi (Neurospora crassa), which used a plug-flow bioreactor (PFBR) to feed the substrate continually and then return a portion of the product back to the bioreactor as inoculum into the system (R. Wang et al. 2021).
In addition to fed-batch and (semi)continuous fermentation for improved productivity and operation costs, processes can include other strategies like media recycling and gas recycling to improve operating cost efficiency and sustainability. As the name suggests, gas recycling is the reuse of exhaust gasses back into the system as gas inputs. This can improve operating costs and efficiencies in gas fermentation systems where the gas feedstock needs to be continuously supplied (Richter, Martin, and Angenent 2013). A gas recycling system has increased equipment needs and operational complexity but can greatly improve gas use efficiency and sustainability.
Media recycling can similarly be implemented to improve feedstock use efficiency and is often applied to (semi)continuous systems where biomass is the final product. After biomass dewatering (removal of water), a portion of the ‘spent’ broth can be returned to the fermentation process since key substrates may still be present at high concentrations. Media recycling also helps to improve the water-use efficiency of a fermentation process. Overall, though, this approach comes with higher operational complexity, increased risk for contamination, inhibitor accumulation, and the potential for reduced productivity depending on the recycle ratio and nutrient replenishment rate.
Process monitoring and control
Bioprocesses must be monitored and controlled over time for maximum productivity. These processes can be monitored on-line or in-line directly connected to the bioreactor, or at-line and off-line outside the bioreactor (Figure 8). Process analytical technology (PAT) allows for bioreactor process control in real time by monitoring the physical, chemical, and biological parameters. This can improve microbial or cell growth, product formation, and yield, and it enables batch-to-batch reproducibility. Overall, PAT allows for critical process parameters (CPP) and key performance indicators (KPI) to be continually monitored and controlled during biomanufacturing (Gerzon, Sheng, and Kirkitadze 2022).
Many types of PAT sensors are used to monitor bioprocesses. These can be grouped into three categories by variable type: (1) physical, such as temperature, vessel pressure, agitation rate, and medium viscosity, (2) chemical, such as pH, nutrient concentration, and gas concentration like dissolved oxygen (DO), and (3) biological, such as biomass concentration and titer (Figure 8) (Biechele et al. 2015). In commercial systems, the most commonly used online or inline sensors are temperature, pressure, pH, and dissolved oxygen probes. Continuous substrate monitoring for nutrients like glucose has been advanced through on-line electrochemical and biosensor monitoring (Pontius et al. 2020). Novel optical sensors, such as UV/Vis, RAMAN, and fluorescence spectroscopy probes, have been major developments in bioprocess monitoring and continue improving the monitoring accuracy of many parameters, such as cell density (Biechele et al. 2015).
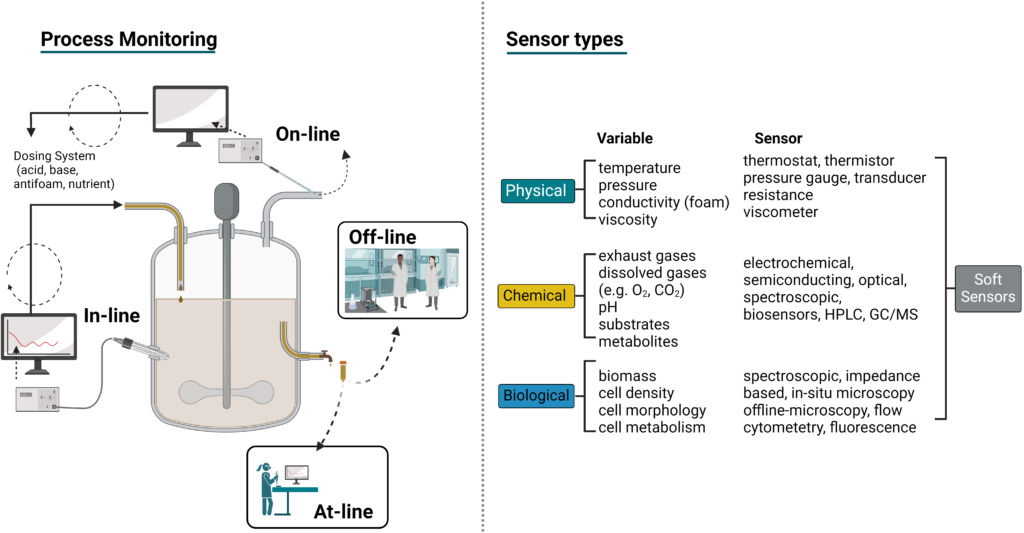
At-line or off-line monitoring builds data knowledge on a process or measures a production run for KPIs by pulling samples and measuring a parameter in the lab. In-line or on-line process monitoring provides continuous, real-time feedback that then allows for subsequent process and KPI control. However, not all target variables can be directly quantified with in-line/on-line sensors during a bioprocess. Software sensors, also called soft sensors, are predictive software models built from bioprocess data sets and hard sensor data that can indirectly quantify challenging target variables (Rösner et al. 2022).
Soft sensors combine previous process input and output data with a model that uses the real-time PAT input data to predict a target output (Brunner et al. 2021). The underlying models can be built from process data using mechanistic models, which have a detailed understanding of physical process mechanics (white box model) or empirical models using simple input and output observation data. Empirical models are data-driven models that can be built out using an artificial neural network (ANN or black-box model). Using ANNs can be especially helpful in bioprocesses where the underlying biological mechanisms are highly complex and difficult to mechanistically model. ANN soft sensors estimate and predict the targeted variable based on the input and output data used for training the model (Rösner et al. 2022). These models require large process datasets to train, need to be retrained at different scales, and have range limitations based on training data (Bolmanis et al. 2023).
Soft sensor models can be especially useful in predicting biochemical parameters for which suitable on-line and in-line sensors do not exist. For example, online intracellular lipid sensors are not yet available, and monitoring microbial cells’ lipid content for fat production requires laborious off-line sample measurement. Wang et al. 2023 used an ANN model to predict lipid content based on proxy parameters like glucose, dissolved oxygen, and base addition. The model was trained on prior process input data (time, DO, glucose, and NaOH) and output data (glucose, offline cell dry weight, and lipid content), which resulted in a soft sensor that could accurately predict lipid content from proxy real-time data (Wang et al. 2023). These models can be adapted to various hard sensor data. In practice, spectroscopic or fluorescence sensors offer a range of data that can be modeled to different biological variables for soft sensor training (Rösner et al. 2022).
The combination of sensors, recorder, set-point, controller, and control element create a control loop, which can be open-loop or closed-loop. Open control loops involve measuring output, such as temperature, with no automatic or feedback control. Open control loops might involve simple human intervention to control the process, such as through manual temperature adjustment or manual agitation changes, which is not an ideal commercial strategy. Open-loop controls can also be used for feeding systems when a precalculated substrate feed rate is provided for a controller to deliver the nutrient to the bioreactor based on that schedule. Feed rates can be set linearly or determined empirically through many cultivations (Bolmanis et al. 2023).
A closed or feedback control loop operates through real-time monitoring and automatic control of a parameter based on the set-point. All closed-loop control systems respond to nonlinear system dynamics with variable control actions. Closed-loop control methods include proportional integral derivative (PID) control and model-predictive control (Bolmanis et al. 2023). A PID controller is the most commonly used closed-loop controller, such as for dissolved oxygen, linked agitation control, or acid-base feeding for pH control. A PID continuously calculates error signal values as the difference between the set-point and the measured process variable. Model-predictive controls (MPC) are very promising methods for fed-batch feeding system controls. MPC integrates a mechanistic or empirical model (such as ANN models) to predict process control feed rates or other output parameters based on measured input reference values (Helleckes et al. 2022).
Model-based systems are gaining popularity for high-throughput screening and bioprocesses, such as PAT soft sensors, digital twins (modeled digital bioreactor), machine learning models, and model-predictive control. All these areas are driven by adoption and advancements in machine learning, which allows for analyzing large, complex datasets that can be difficult to model mechanistically. These models and process control techniques aim to increase and predict production titers, rates, and yields (TRY) (Helleckes et al. 2022). While process control has advanced, it’s important to screen and assess bioprocess at various scales and expected conditions, especially for model training at a commercial scale.
Scaled-up and scaled-down bioprocess testing
Bioprocesses need to be incrementally scaled from lab (0.25-10 L) to pilot (25-1000 L) to demonstration (1-25 m3) to commercial scales (25-1000 m3) to assess and optimize commercial conditions as well as assess the economic viability of the process. Despite progress in modeling and software approaches, there is no substitute for an integrated pilot process. Many processes are fit for purpose, which requires testing at scale to understand yields and areas for improvement.
After the lab scale, bioprocesses are first assessed in a pilot system with scale-matched DSP to translate the lab-scale process into a scaled-down commercial manufacturing process. After the pilot, the bioprocess is further scaled to a demonstration scale to assess the techno-economics of the process at even larger volumes, which helps to de-risk the large capital investment required for full commercial scale. Companies can either build pilot and demonstration facilities or utilize contract manufacturers (or toll manufacturing) for demonstration. See our manufacturing capacity landscape for more on scaling up a commercial process.
It’s important to consider the commercial-scale production conditions when optimizing the bioprocess. This can be accomplished via scaled-down testing throughout the scale-up process. Fermentation bioprocesses have complex system operations with many parameters impacting performance and likely changing during volumetric scaling. Scaled-down testing assesses a biological process at lab and pilot scale under commercially relevant conditions, feedstocks, and unit processes (Tajsoleiman et al. 2019).
The objective of scaled-down testing is to maintain physical and physiological similarity between large and small scales. These conditions can be mimicked/modeled, to an extent, at lab scale to assess productivity. Examples of scale-dependent parameters include differences in feedstock quality from lab to commercial, vessel hydrostatic pressure differences, changes to maximum gas-liquid mass transfer, and mixing differences at scale (Crater and Lievense 2018). See Table 2 for a list of common scale-dependent parameters and their implications. It’s important to understand and expect productivity changes at the commercial scale due to scale-dependent factors. Moreover, assessing commercially relevant conditions in scale-down testing and techno-economic models based on productivity results can reduce scaling risk.
Additionally, mimicking fermentation conditions in strain screening protocols will ensure that selected strains ultimately perform as expected in commercial conditions (i.e. “you get what you screen for”). Strains can be initially screened in high throughput methods (i.e. plates, flasks) in relevant conditions and then scaled to small bioreactors for further scaled-down productivity assessment.
Parameter | Deviation | Impact |
---|---|---|
Feedstock quality | Grade (industrial vs reagent), purity, concentration, variability | Impurities and unfermentable components can impact titer, rate and yield |
Feedstock and medium sterilization | Batch vs continuous sterilization (lab vs commercial) | Sterilization mode may impact the stability or degradation of medium components |
Mixing | Increased mixing time or decreased homogeneity | Lower productivity due to gradients that may form for parameters like temperature, pH, DO, and substrate |
Gas-liquid volumetric mass transfer | Changes in the gas distribution or mixing due to equipment and cost limitations | Slower or unequal gas distribution leads to lower productivity |
Liquid hydrostatic pressure | Large vessel increases pressure gradient | Increased gas concentrations impact productivity and cell stress |
Shear stress | Increased shear in some conditions to maintain mixing | High shear stress affects cell morphology, productivity, or DSP yield |
Commercial case studies
This deep dive discusses upstream bioprocesses from reactor type, growth, cultivation modes, process control, and more. But what does an upstream bioprocess look like commercially for those already producing food products? Many companies are employing numerous strains, medium formulations, bioreactor types, and cultivation strategies. Below are a few commercial examples that implement the above upstream process concepts.
The “simple case” of baker’s yeast
Let’s start with simple baker’s yeast (Saccharomyces cerevisiae). This yeast strain is valued for its dough-leavening ability and has been used extensively in beer and bread production. Commercial fermentation of baker’s yeast to produce a compressed, dried, or instant biomass was established to meet the growing demand of the baking industry. The fermentation typically occurs in a few 100 m3 large-scale aerobic bubble column fermenters with molasses as the substrate (Paulová, Patáková, and Brányik 2013; Noorman and Heijnen 2017).
The process should be simple to produce yeast biomass. However, due to the ‘Crabtree effect’, which forms alcohol under aerobic conditions in the presence of excess substrate (i.e. glucose), the process must use a fed-batch strategy to control glucose inflow to balance biomass generation and prevent alcohol formation (Paulová, Patáková, and Brányik 2013). Maintaining the dissolved oxygen and precisely feeding the molasses substrate in a fed-batch mode requires tight process control and feeding regiment. This can be controlled through empirical feed-forward control strategies or by implementing process sensors that are tied to the substrate and dissolved oxygen concentrations.
The fed-batch baker’s yeast process is limited by mixing and sufficient oxygen transfer at large scales when trying to maximize biomass production versus alcoholic fermentation (H. J. Noorman et al. 2018). Biomass yields can easily be reduced by 5-10% upon commercial scale-up, which is a significant hit in productivity for a process with tight margins. This is why even simple baker’s yeast has been tested under scaled-down conditions for many decades to mimic commercial conditions, such as mixing, glucose gradients, and oxygen concentration, and to assess optimal operating conditions like fed-batch feeding regimes.
Filamentous fungi in airlift bioreactors
Filamentous fungi are well known for producing antibiotics, organic acids, enzymes, and recombinant proteins. A well-known food application of filamentous fungi is the protein-rich mycoprotein produced by Marlow Foods (Quorn), which has a fibrous texture that mimics meat. Today, Quorn’s mycoprotein is sold as burgers, sausages, and other alternative meat products, and is produced through their submerged fermentation process.
In the Quorn process, the filamentous fungi Fusarium venenatum is cultivated in a 155 m3 external-loop ALR using a continuous flow culture process designed by Marlow Foods (Figure 9). The external-loop ALR design has several advantages for the Quorn process. At large scale the ALR, versus a stirred tank, allows for more efficient OPEX costs and is gentle on the filamentous fungi hyphae, which help create the meat-like fibrous texture. If grown in an STR, the rotating blades and high shear would damage the hyphae. Additionally, the external-loop design provides improved liquid flow and mixing of fresh substrate (glucose and ammonium), which enters at the bottom of the reactor and circulates with the cell suspension through the upward rise of the gas. Finally, the tall liquid height causes immense hydrostatic pressure at the bottom of the bioreactor, allowing oxygen to dissolve into the medium quickly. The low pressure at the top of the reactor riser allows carbon dioxide waste to degas and exhaust before the culture returns through the downcomer (Moore, Robson, and Trinci 2019).
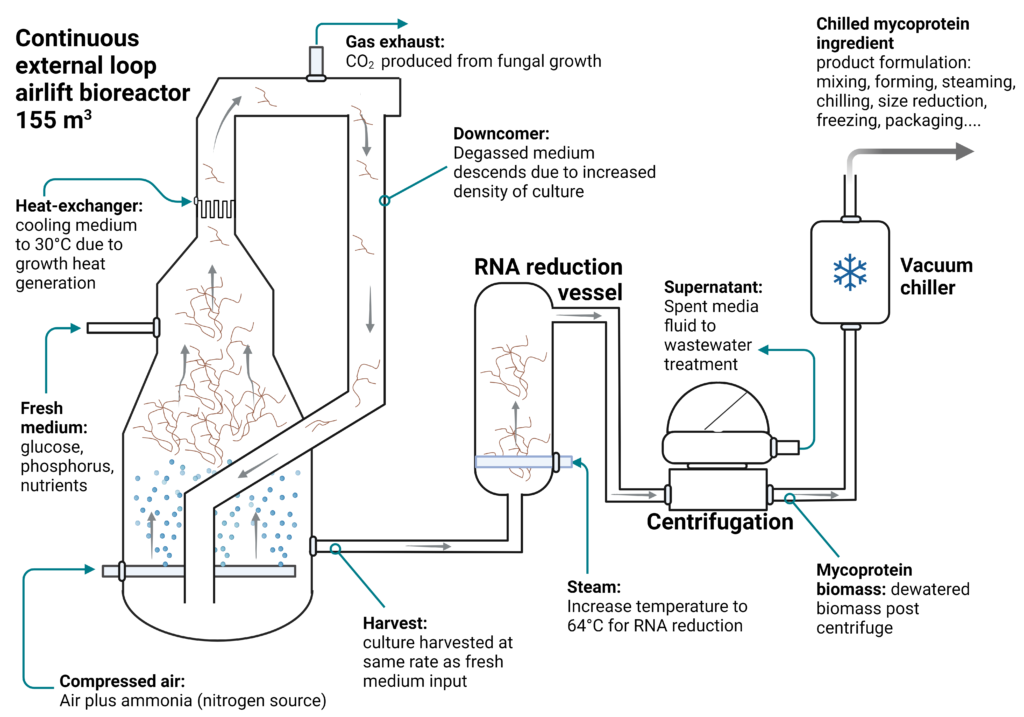
Quorn’s continuous culture process allows for higher productivity versus a batch culture, and is one of the largest continuous systems in the world (Moore, Robson, and Trinci 2019). The fermenter is inoculated with low-density Fusarium venenatum culture, which rapidly grows and enters the continuous cultivation phase after only four days of initial growth. The process runs continuously for up to 1000 hr (6 weeks), after which the process is terminated and the system cleaned and reset for another run. While the continuous culture could keep growing, the Quorn process is terminated due to the accumulation of highly branched mutant strains of the F. venenaturm. While these mutants are nutritionally identical, their morphology impacts the desired final texture of the mycoprotein meat alternative (Moore, Robson, and Trinci 2019).
During the continuous culture, carbon as glucose is delivered through fresh medium input, and nitrogen as ammonia is supplied through the gas inlet with the compressed air input. Delivery of ammonia (a weak base) is regulated through a pH feedback control loop to maintain a culture set-point pH. Glucose is added during continuous cultivation by maintaining the culture in a chemostat system with a biomass concentration of 10 – 15 g L-1. In the chemostat process, biomass is removed and fresh medium with glucose is added at a constant rate of 0.17-0.2 hr-1, which equates to a 30,000 L hr-1 or 375 kg-biomass hr-1 output rate. The harvested biomass is then sent to a DSP that includes a heat treatment for RNA reduction followed by dewatering (Moore, Robson, and Trinci 2019).
The Quorn process is a technologically mature bioprocess and the result of over thirty years of R&D and commercial production. ENOUGH (formerly 3F Bio) produces a mycoprotein product (ABUNDA) from a similar process and the same strain of fungi, Fusarium venenatum. Their process uses a modified submerged fermentation process using hydrolyzed starch glucose feedstock and incorporating medium recycling after each continuous harvest. Recycling the water and leftover glucose in the medium further improves the economics and sustainability of the process. Additionally, their co-location with Cargill’s starch facility in the Netherlands improves glucose feedstock availability and reduces transportation costs.
Gas fermentation: single-cell protein production
A promising fermentation technology is gas fermentation, which uses gasified feedstocks for microbial bioprocesses. This includes C1 carbon gasses like methane (CH4), carbon monoxide (CO), and carbon dioxide (CO2). There is a growing and evolving gas fermentation sector for biomass and biochemical production due to the sustainable process potential by using gas feedstocks versus organic sugar-based fermentation, which can have a higher environmental impact over gas feedstocks and may pull from food crops. One area of commercialization is the production of microbial biomass, also called single-cell protein (SCP), through gas fermentation bioprocesses.
SCP production has strong potential as both an end-product food and feed source. Gas fermentation companies like Solar Foods and Air Protein are using hydrogenotrophs (hydrogen oxidizing bacteria) to produce meat substitutes for human consumption (Marcellin et al. 2022). By contrast, companies like Unibio and Calysta are commercializing SCP production for feed production using the methylotrophic bacteria Methylococcus capsulatus to convert methane to protein. These companies are also looking to expand into the human and pet food sectors using this technology. While methane-based processes currently use natural gas for feed production, the process could also be run off methane sourced from waste anaerobic digesters (Jain et al. 2023).
The process deployed by Unibio and Calysta implements the U-loop style bioreactor, continuous fermentation, and medium recycling operation mode (Figure 10) (Olsen et al. 2020). These processes are crucial for high bioprocess output and sustainability. The U-loop bioreactor with counter-current gas delivery and highly pressurized headspace helps to increase gas mass transfer due to the low solubility of the methane or other C1 gasses by driving the gas bubbles down into the loop (Banks et al. 2022; Marcellin et al. 2022). In the U-loop SCP process, methane, oxygen, ammonia, and minerals are added to the bioreactor in a chemostat mode to maintain biomass at a steady state (~20 g L-1 ) while it’s continuously harvested (Olsen et al. 2020). The harvested biomass is dewatered by a centrifuge, concentrated by ultrafiltration, then homogenized and dried to create the feed protein. The water separated during the DSP is also recycled back into the fermenter to reduce water and nutrient loss during the process. Theoretically, the gas exhaust waste can be recycled back into the reactor or used as an energy source for either steam boilers or spray dryers, since some of the methane likely is unused in the loop (Banks et al. 2022; Marcellin et al. 2022).
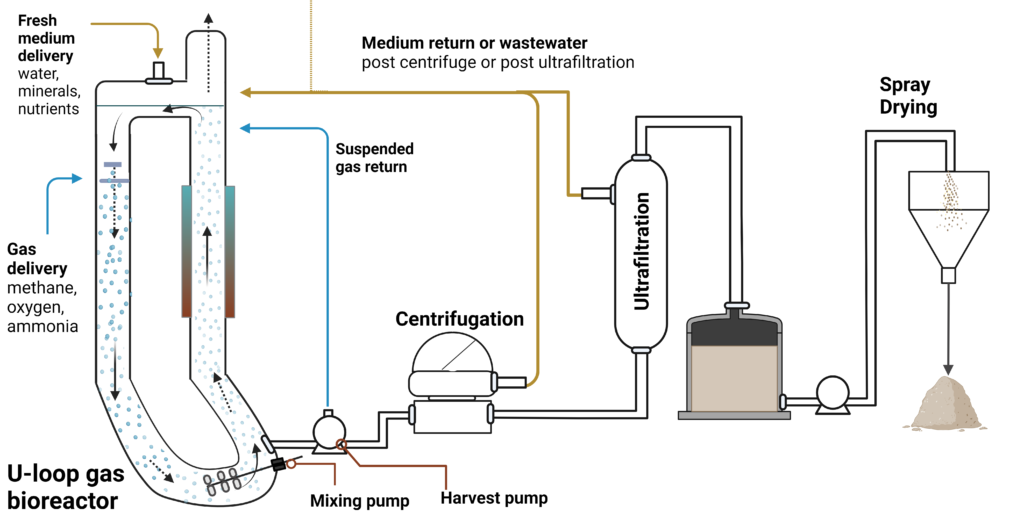
The mighty yet simple tray reactor: Solid-substrate or solid-state?
The most commonly used solid-state reactor system for edible fungi is the tray reactor described above. For traditional koji production, this typically takes place in a climate-controlled room where steamed rice inoculated with Aspergillus orzyae (koji spores) is incubated in a chamber and then transferred to trays with ventilated systems. These trays then require intermittent manual mixing since the koji will heat up in the middle of the bed due to microbial metabolism. Modern-day koji production reduces manual labor by incubating in static flatbed reactors with agitators or by using larger SSF commercial systems with advanced air handling, process control, mechanical mixers, and sanitation systems like rotary-drum reactors or stirred bed reactors.
Several mycelial fungi alternative protein companies, such as MyForest Foods and Nature’s Fynd, have adapted the traditional tray systems for mycelial biomass production. As mentioned, SSF suffers from a number of disadvantages, such as the produced biomass being tightly entwined with the solid substrate, making separation difficult if feedstock material is undesirable in the final product. To address this, Nature’s Fynd doesn’t use a solid substrate in their tray reactor system. Their process uses a surface liquid fermentation method that they call liquid-air interface fermentation (LAIF) adapted for mycelial mat production in solid-state tray reactor systems (Kozubal, Macur, and Avniel 2022).
In Nature’s Fynd’s patented process, a liquid growth medium is inoculated with filamentous fungi, such as Fusarium novum yellowstonensis, Fusarium venenatum, or Rhizopus oligosporus, and then incubated in a controlled chamber until a thick fungal biomat forms (Kozubal, Macur, and Avniel 2022). This LAIF process has inherent advantages over traditional SSF in harvesting, quality control, and contamination control. The liquid medium can undergo a typical batch sterilization process before inoculation, and the medium is acidic, which reduces opportunistic microorganism contamination during biomat growth. The trays are incubated on a tray rack in a chamber until the biomats reach a density of 50 – 180 g-biomass L-1 media and are 1-33 mm thick. Nature’s Fynd has found that incubation at a liquid air-interface method produces fungal biomats that have more dense, tensile, and long-aligned filaments than via SSF (Kozubal, Macur, and Avniel 2022).
MyForest Foods (formerly AtLast and a spin-off of Ecovative Designs) is another company using a tray system to produce a mycelial bacon alternative called ‘MyBacon’. Their process requires a substrate, a fungus (Pleurotus ostreatus, the species that produces oyster mushrooms), and a growth environment optimized to produce mycelium with a meat-like texture. They improved upon the tray system by creating a patent-pending incubation environment that maintains moisture, oxygen, and carbon dioxide concentration to grow a mycelium mat on a sterilized substrate that can be solid and/or liquid.
In the growth chamber, the moisture content is controlled by a humidity sensor and misting system delivered through the top of the chamber and recycled back from the bottom (Winiski et al. 2022). The process analytical controls also maintain oxygen and carbon dioxide concentrations in the chamber. Maintaining oxygen levels improves aerobic metabolism, while higher than ambient carbon dioxide levels (2-7%) modulate aerial growth on the tray top and prevent fruiting body (mushroom) formation. Fungal hyphae often grow toward areas of high carbon dioxide, typically an indicator of decomposing organic matter in nature, while fruiting bodies form in areas of low, ambient carbon dioxide. Finally, airflow across the top of the trays promotes heat exchange as well as lateral rather than downward growth of the fungal hyphae (Winiski et al. 2022). This example highlights how even the “simple” tray bioreactor can be modified to control the desired growth of a mycoprotein product.
Precision fermentation: Protein production in Komagaetella phaffii
Impossible Foods was the first to introduce a plant-based burger with an ingredient derived from precision fermentation. Leghemoglobin H (LegH) is a soy protein, expressed in roots, that binds to heme and when added to food creates an organoleptic experience that mimics the myoglobin-heme complex in animal muscle protein. To produce LegH, Impossible Foods engineered the budding yeast Komagaetella phaffii (formerly called Pichia pastoris) to produce LegH and the concomitant upregulation of heme biosynthesis. K. phaffii is a workhorse biotechnology microbe also used by TurtleTree, The Every Company, ReMilk, Vivici, and Oobli to produce food proteins (Eastham and Leman 2024). Originally isolated for its ability to metabolize waste methanol from the petrochemical industries, it was soon demonstrated to produce impressive quantities of recombinant protein, such as human insulin for diabetes (Cregg et al. 2000; Cereghino and Cregg 2000; Polez et al. 2016). Indeed, K. phaffii is an important chassis organism for protein expression across biotechnology industries.
While many aspects of these companies’ bioprocess are confidential, the general framework for USP of recombinant protein production in K. phaffii is very well-characterized, as are the general growth requirements of the yeast. Temperatures from 28-30ºC are preferred by most K. phaffii strains, along with pH 5 to 6, and the presence of molecular oxygen diffused in the liquid substrate. Most importantly, a high-cell density fermentation (HCDF) is a key strategy for growth and recombinant protein production in production using K. phaffii, which is accomplished through a fed-batch submerged fermentation process. The production batch can be divided into two phases: (1) glycerol batch phase and/or fed-batch phase, and (2) methanol induction fed-batch phase for protein production.
In this strategy, K. phaffii cells are grown to high density using a feedstock, such as glycerol, that is fed-batch dosed until the cells reach the desired peak production density. Scale-up production begins with stored strains held in frozen glycerol stocks at -80ºC. Next, successive seed trains from frozen stock-inoculated shaker flasks to thousands of liters has been described, with initial reactor volumes and inoculums determined during process scale-up (Liu et al. 2016). The goal is to achieve HCDF in the production bioreactor. Typically, in the bioreactor, an initial bolus of glycerol-containing medium is provided, and at a predetermined time and cell concentration, a feeding rate of glycerol is established. Once the desired cell concentration is achieved, the proliferation stage is completed.
Once HCDF is achieved, the high number of cell factories can then be triggered, known as induction, to produce the engineered protein of interest, which in the Impossible Foods’ case is LegH (Trinh, Ngo, and Truong 2023). In K. phaffii, the cellular induction is triggered by switching the carbon feedstock from glycerol to methanol, which induces the pAOX1 promoter that has been tied to the expression of the recombinant protein. For some strains this shift occurs gradually, but depending on the genetic modifications, for others it can be accelerated by adding methanol directly. For example, in Mut- strains, the methanol does not provide a carbon source for cell growth and proliferation ends; however, the presence of methanol activates the Mxr1 transcription factor that triggers promoters, pAOX1 and pAOX2. Genes driven by this promoter are activated efficiently and the proteins are produced, such as in the heme biosynthesis.
All phases of growth must have optimized feeding controls based on biomass production so that typically there is no residual glycerol remaining during the induction phase. The methanol fed-batch induction and production must be optimized to maintain high protein productivity. Integrated sensor systems can help improve phase monitoring and soft sensors have been developed for K. phaffii to optimize production (Brunner et al. 2020). Moreover, the metabolism of glycerol (and methanol) occurs only in the presence of oxygen, so the bioreactor oxygenation/aeration and the mixing systems must be optimized to provide and distribute oxygen to all of the cells in the system while preventing overly aggressive mixing that may lead to cell shear stress and lysis.
In Impossible Food’s process, the products are accumulated intracellularly and are thus protected from the high-oxygen environment of the extracellular medium and any proteases produced by the yeast. However, extracellular secretion can be desirable in many bioprocesses to streamline downstream processing. Once the production of LegH has been determined to be sufficient, such as through off-line laboratory testing or on-line production sensors (Shao et al. 2022), the USP can be ended and the cells containing LegH can be removed from USP for downstream processing of the LegH-heme complex.